Thank you for visiting nature.com. You are using a browser version with limited support for CSS. To obtain the best experience, we recommend you use a more up to date browser (or turn off compatibility mode in Internet Explorer). In the meantime, to ensure continued support, we are displaying the site without styles and JavaScript.
- View all journals
- My Account Login
- Explore content
- About the journal
- Publish with us
- Sign up for alerts
- Open access
- Published: 02 December 2016

Learning by Association in Plants
- Monica Gagliano 1 ,
- Vladyslav V. Vyazovskiy 2 ,
- Alexander A. Borbély 3 ,
- Mavra Grimonprez 1 &
- Martial Depczynski 4 , 5
Scientific Reports volume 6 , Article number: 38427 ( 2016 ) Cite this article
97k Accesses
128 Citations
781 Altmetric
Metrics details
- Behavioural ecology
- Evolutionary ecology
In complex and ever-changing environments, resources such as food are often scarce and unevenly distributed in space and time. Therefore, utilizing external cues to locate and remember high-quality sources allows more efficient foraging, thus increasing chances for survival. Associations between environmental cues and food are readily formed because of the tangible benefits they confer. While examples of the key role they play in shaping foraging behaviours are widespread in the animal world, the possibility that plants are also able to acquire learned associations to guide their foraging behaviour has never been demonstrated. Here we show that this type of learning occurs in the garden pea, Pisum sativum. By using a Y-maze task, we show that the position of a neutral cue, predicting the location of a light source, affected the direction of plant growth. This learned behaviour prevailed over innate phototropism. Notably, learning was successful only when it occurred during the subjective day, suggesting that behavioural performance is regulated by metabolic demands. Our results show that associative learning is an essential component of plant behaviour. We conclude that associative learning represents a universal adaptive mechanism shared by both animals and plants.
Similar content being viewed by others
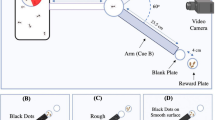
Using pupae as appetitive reinforcement to study visual and tactile associative learning in the Ponerine ant Diacamma indicum
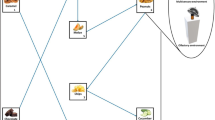
Human spatial memory implicitly prioritizes high-calorie foods
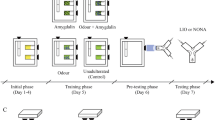
Young honeybees show learned preferences after experiencing adulterated pollen
Introduction.
The ability to choose among different and often conflicting options, and predict outcomes, is a fundamental aspect of life 1 , 2 , 3 , 4 . One form of choice behaviour is based on establishing an association between an occurrence of external events and the opportunity to satisfy internal homeostatic needs, such as hunger, thirst or sleep. The notion that choices are driven by the expectation of their rewarding outcome goes back to Aristotle 5 and has been observed extensively across the animal kingdom 6 , 7 , 8 , 9 . However, it remains unknown whether this is also true for plants.
In the complex photosynthetic world of plants, light plays an especially important role in growth and survival. Its role is dual. On the one hand, light energy is necessary for processes of biosynthesis. On the other hand, light provides a time cue for entrainment of the circadian rhythm to the 24-h cycle, thereby optimizing the adjustment of growth and metabolism to the seasonal variation of the photoperiod 10 . Therefore, the ability to detect salient cues that increase efficiency in foraging for light is absolutely essential and confers a significant evolutionary advantage. Plants have recently been found to acquire new behaviours to enhance foraging efficiency for light through the non-associative learning process of habituation 11 , and thus to facilitate photosynthesis and growth. However, it remained unknown whether plants can also learn through forming associations.
To investigate this possibility, we employed a classical conditioning paradigm where a neutral environmental cue (a conditioned stimulus, CS) predicted the occurrence of light, which is biologically significant (an unconditioned stimulus, US). In the first experiment, pea seedlings (n = 45) were entrained to an 8-h light:16-h dark cycle for 5–8 days. In the subsequent 3-d training period, they were kept in darkness with the exception of 1-hour light exposures during the three daily training sessions. Training occurred individually inside a Y-maze, where the airflow produced by a fan ([F] as the CS) and a blue LED light ([L] as the US) were systematically presented according to a specific protocol ( Fig. 1 and details in Methods section; see also Extended Data Fig. 1 and Supplementary Video 1 ).
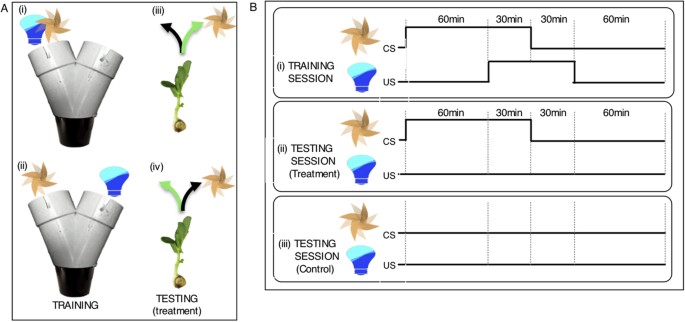
Training and testing protocol for associative learning in pea seedlings.
( A ) During training seedlings were exposed to the fan [F] and light [L] on either the same arm (i) or on the opposite arm (ii) of the Y-maze. The fan served as the conditioned stimulus (CS), light as the unconditioned stimulus (US). During testing with exposure to the fan alone two categories of responses were distinguished. Correct response : Seedlings growing into the arm of the maze where the light was “predicted” by the fan to occur [green arrow; iii (corresponding to scenario i) and iv (corresponding to scenario ii)]; Incorrect response: Seedlings growing into the arm of the maze where the light was not “predicted” by the fan to occur (black arrow; iii and iv). ( B ) Seedlings received training for three consecutive days before testing. Each training day consisted of three 2-h training sessions separated by 1-h intervals. The 90-min CS preceded the 60-min US by 60 minutes so that there was a 30-min overlap. (i). During the 1-day testing session, seedlings were exposed to the fan alone for three 90-min sessions (ii). Seedlings of the control group were left undisturbed (no fan, no light; iii).
Prior to training, seedlings were randomly assigned to one of 2 experimental groups. In one group exposure to the light and fan was on the same arm of the maze [F + L], whereas in the other group light and fan were on opposite arms [F vs L] ( Fig. 1Ai,ii ). Accordingly, this design tested for both a positive association of the fan (CS) with light (plant trained to seek out fan as a predictor of light) and a negative association of the fan with light (plant trained to avoid the fan and find light on the side of the tube with no air movement). The protocols were maintained throughout the 3-d training period. However, to render the direction of the incoming light unpredictable, its position with respect to the arm of the maze was re-assigned for each 120-min training session (details in Methods section). During training, the seedlings grew and approached the Y-bifurcation of the maze.
Before the testing day, the seedlings were further subdivided randomly into a test group (n = 26) and a control group (n = 19; the numbers are unequal due to a technical problem). The test group was exposed only to the fan during the three 90-min sessions. In this group, to control for the influence of innate phototropic response, the fan was placed in the arm opposite to last light exposure in the [F + L] group and on the arm of last light exposure in the [F vs L] group. The seedlings of the control group were left undisturbed. On the morning after the testing day, we visually inspected the seedlings and recorded the arm of the maze they had grown into ( Fig. 1Aiii,iv ).
As expected, we found that all seedlings of the control group grew into the arm of the maze where the blue light had been presented in the last training session (white bars; Fig. 2 ). This result corroborates the well-known innate phototropic response of seedlings to blue light 12 . In contrast, in the test group, the majority of seedlings exhibited a conditioned response to the fan (green bars; Fig. 2 ). In the [F + L] group, 62% of the seedlings grew towards the fan ( Fig. 2A ), whereas in the [F vs L] group, 69% of the seedlings grew in the direction opposite to the fan ( Fig. 2B ). Thus, the first experiment has shown that plants are able to form associations to enhance foraging success.
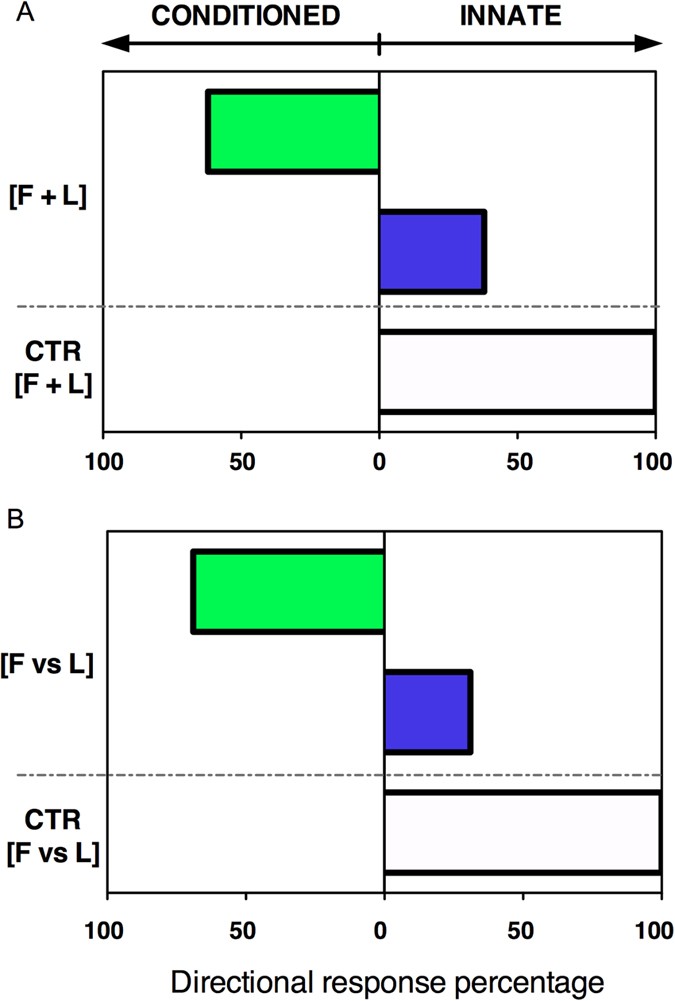
Associative learning in pea seedlings.
In the absence of the fan, all control seedlings (100%) directed their growth toward the arm of the maze where the light was last presented (white bars). In the presence of the fan, the majority of seedlings grew toward the arm of the maze that had been associated with light during training ([F + L]: same side; [F vs L]: opposite side), thus exhibiting the conditioned response (green bars). A smaller proportion of seedlings did not show learning, thus exhibiting the innate response (blue bars). The response of the experimental groups was significantly different from controls (Two-tailed Fisher’s Exact Test, P = 0.0027 for [F + L] and P = 0.0017 for [F vs L]). See Data file in Supplementary Information .
In animals, the circadian system provides a framework for a wide range of behaviours. The ability to anticipate changes in food availability enables efficient interactions of the organism with the environment in a time of day dependent manner, and facilitates learning 13 , 14 . Great progress has been achieved in characterizing the molecular and physiological basis of circadian rhythmicity in plants 15 . However, it remains unknown whether the time of day modulates behavioural processes such as learning, in plants.
In our second experiment, seedlings (n = 83) were trained and tested inside a Y-maze, where temperature and light served as Zeitgebers 16 ( Fig. 1B ; details in Methods section). The training and testing procedure corresponded to that of the first experiment. However, exposure to fan and blue light occurred always on the same arm of the maze ([F + L] condition). The main variable was the phase of the 24-h temperature cycle in which the training and testing sessions occurred.
Prior to training, the seedlings were randomly assigned to one of 3 experimental groups. They were maintained under controlled environmental conditions (12-h light:12-h dark coinciding with high-low temperature 21 °C:17 °C). The temperature cycle served as the Zeitgeber that was maintained throughout the training and testing periods. The timing of the experimental ‘day’ (light +21 °C) in the growth chamber varied between the groups ( Fig. 3A ): Group 1 experienced ‘day’ from 07:00–19:00 (‘Light’), Group 2 from 01:00–13:00 (‘Light-Dark’) and Group 3 from 19:00–07:00 (‘Dark’). After emergence, each seedling was transferred into its individual Y-maze where one of the arms was used to deliver both the unconditioned stimulus (light, US) and the conditioned stimulus (fan, CS). Since both [F vs L] and [F + L] protocols had been equally effective in the first experiment, only the latter protocol was used here. During the three training days, the seedlings were maintained at their respective temperature regimes in total darkness, except for the three 1-h sessions with blue light exposure paired with the fan (see orange and blue rectangular areas; Fig. 3A ). These sessions occurred at the same external clock time in the three groups (10:00, 13:00, 16:00), but at different phases of the 24-h temperature cycle. On the testing day, only CS was delivered in the three 90-min sessions ( Fig. 1Bii ; see orange rectangular areas only, Fig. 3A ). The control groups were left undisturbed on the testing day ( Fig. 1Biii ).
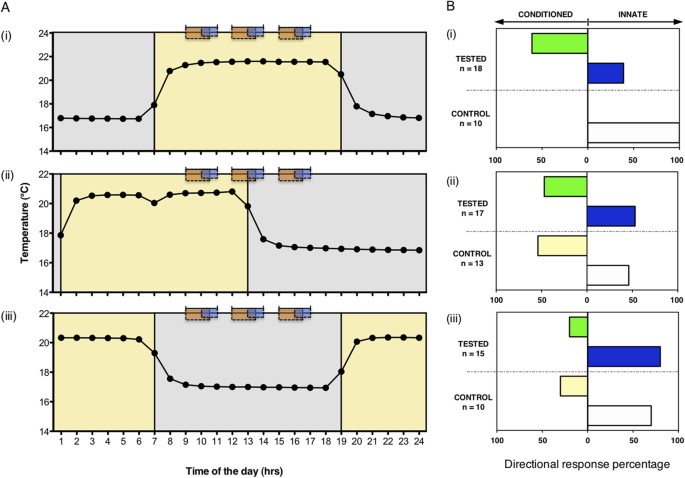
Circadian effects on behavioural performance of pea seedlings.
( A ) Seedlings were kept in incubation chambers, where initially both light and temperature were used as Zeitgebers (temperature = dotted line; mean values across all days; light:dark cycles, yellow:grey shaded areas). During three training days, the seedlings were kept in darkness with the exception of the three training sessions, while the temperature cycle was maintained (note: the LD cycle was not maintained during training). The training (orange and blue rectangular areas, indicating the time of exposure to the fan and the blue light respectively) and testing sessions occurred during the former light phase in the Light group (i), and partly or entirely outside the former light phase in Light-Dark (ii) and Dark group (iii), respectively. ( B ) In the ‘Light’ group (i), the growth response of tested seedlings was significantly different from control seedlings (Two-tailed Fisher’s Exact Test, P = 0.002). All control seedlings grew to the arm of the maze where the blue light had been delivered on the last training day [white bar; (i)], while 61% of tested seedlings grew towards the arm where the fan predicted the blue light to occur [green bar; (i)]. A minority of tested plants (39%) did not form an association [blue bar; (i)]. Under phase-shifted conditions, the tested seedlings did not differ from controls [Two-tailed Fisher’s Exact Test, P = 0.769 for the Light-Dark group (ii); P = 0.653 for the Dark group (iii)]. Phase-shift disrupted the phototropic response of control seedlings [white bars; (ii, iii)], causing only 46% of individuals in the Light-Dark group and 70% in the Dark group to direct their growth towards the side of last light exposure [white bars; (ii, iii)].
In the ‘Light’ group, the majority of tested seedlings grew towards the fan, opposite to the arm where the light was delivered during the last training session (green bar; Fig. 3Bi ), confirming the results of the first experiment. All control seedlings in this experimental group grew towards the arm of the last light exposure (white bar; Fig. 3Bi ), also confirming previous findings. However, this was no longer the case in the ‘Light-Dark’ and ‘Dark’ group, in which training and testing occurred at different phases of the temperature cycle. Under these conditions, learning was not successful (47% and 20% in the ‘Light-Dark’ and ‘Dark’ group respectively; green bars; Fig. 3Bii,iii ). Growth towards previous light exposure of undisturbed control seedlings was reduced in the ‘Dark’ Group (white and yellow bars; Fig. 3Biii ), and abolished in the ‘Light-Dark’ group. In the latter, the innate phototropic response, which is consistently 100% efficient when exposure to light occurs during daylight hours (white bars; Figs 2 A,B and 3 Bi), is no longer displayed and growth behaviour appears random (white and yellow bars; Fig. 3Bii ).
Thus, our results show that pea seedlings develop an association that facilitates growth towards the light based on the occurrence of a neutral cue. This learned behaviour prevails over innate positive tropism to light, which is thought to be the major determinant of growth direction in plants. In both experiments, the ability of seedlings to anticipate both the imminent arrival of light (“when”) and its direction (“where”) based on the presence and position of the fan indicates that plants are able to encode both temporal and spatial information and modify their behaviour under the control of environmental cues. This form of learning is ubiquitous in the animal kingdom 17 , 18 , including all major vertebrate taxa and several invertebrate species 19 and can also be implemented in artificial networks and machines 20 . Whilst the possibility that plants also learn by association has been considered by earlier studies 21 , 22 , our current study provides the first unequivocal evidence.
The learning paradigm characterised here opens new and exciting opportunities for examining numerous other forms of associative learning mechanisms as well as phenomena that arise within associative learning in plants. For example, our current experimental design could be easily extended by including an experimental condition, where the US precedes (and then overlaps) the CS. If this too elicits the same conditioned response as CS preceding US, this would provide evidence for a form of associative learning known as conditional sensitization. Similarly by extending our design to test different temporal arrangements between the CS and US in learning acquisition (e.g. the CS and US are presented at widely separated intervals and/or in alternate orders), the role of timing and how spatiotemporal relationships between events are encoded within an association in plants could be readily assessed and then compared to findings in animal studies 17 , 23 . Additionally at the ecological level, it would be most interesting to test whether/how plants respond to other, novel neutral stimuli (besides the fan used in our study); this would provide evidence for stimulus generalization, another form of associative learning that occurs when, after a conditioned response has been established to a particular CS, other similar stimuli to the CS will elicit the same response. Because the ability to generalize previous learning and apply it to novel situations endows organisms with the behavioural flexibility to adjust to environmental change without having to learn ‘from scratch’ in each specific situation 24 , 25 , this aspect of associative learning is of great ecological relevance. Accordingly, we believe that experimental efforts directed towards its study under natural conditions could make important contributions to our understanding of plant ecology.
Besides opening up new experimental research avenues, our findings raise some obvious questions about the underlying physiological/molecular mechanisms by which plants can integrate environmental and internal cues and coordinate complex patterns of information during associative learning, so that a more effective, even adaptive, behavioural response can be expressed. At the molecular level, the role of epigenetic reprogramming has been identified as a potential candidate mechanism underlying learning processes across taxa 26 , 27 , including plants 11 , 28 . In multicellular organisms with a nervous system, changes in the synaptic strength between neurons, for example, can be stored as a memory trace that sustain associative learning. In plants and other organisms that do not have a nervous system, modifications of the patterns of interactions between molecules and communication between cells can be stored in a way rather similar to neural networks 29 . Presumably, then, the mechanisms maintaining associative learning operate in plants as in other organisms on the basis of fundamental ‘rules’ that alter the flow of information by modifying the shape and connections within a network via epigenetic changes 26 . While the specific mechanisms that underlie associative learning in plants are to be defined and may be quite different from other system, comparative studies of the molecular tools involved in associative learning across the different systems could be useful in revealing such fundamental ‘rules’. Research in invertebrate animals such as Aplysia , for example, represents a remarkable success story and an example of how using a simple behaviour and a non-physiological manipulation resulted in fundamental discoveries going far beyond a specific learning paradigm and species 30 , 31 .
While the mechanisms underlying associative learning in plants remain to be determined, there is little doubt that it is likely to enhance opportunities to locate and capture available light in the environment. It has been shown that auxin signalling systems may be involved in the integration between photo- and mechanosensory information, which enables directional growth to optimize photosynthesis 32 , 33 . Earlier experiments have demonstrated that young plants can form stable memories pertaining to the direction of a light source 34 , while a more recent study showed that plants optimize opportunities to access and forage for light by learning to ignore recurrent, but unimportant stimuli in their environment 11 . Our results extend these findings by showing that plants are able to adapt quickly to changes in the environment and develop anticipatory behaviour, which may play an important role in maintaining metabolic homeostasis.
Consistently, we show in the second experiment that associative learning occurs most readily during the subjective day. This experiment, in which plants were trained at three different time periods within 24 hours, revealed that the learning effect disappears when training occurred during the evening hours when light would not normally be available. This finding is particularly intriguing and bolsters the argument that associative learning is an adaptive response that is only utilized during daylight hours (when it is most useful) via an internal circadian clock. Interestingly, the response of the control group in this experiment was affected by the phase of the temperature cycle. While in the Dark group the majority of seedlings still grew towards the side of the last light exposure, this was not the case for the Light-Dark group. Thus the phototropic response which was 100% efficient in the Light group, proved to be attenuated or abolished in the other groups. Therefore, the main finding of the second experiment is that phototropism of peas is circadian phase-dependent. This could be due to a modulation of light-sensing capacity or of the transmission of light-induced processes to structures affecting growth (see ref. 35 for review of the topic). Given that alignment of the internal circadian phase with external environmental signals is essential to maintain efficient photosynthesis during the day as well as optimal utilization of reserves during the night 36 , plants are likely to incur an energetic cost when performing light-foraging behaviours at a time that is misaligned with the internal circadian signals. Our results suggest that under these conditions, the expression of both innate and learned behaviours is traded off to meet basic metabolic demands in order to ensure growth and survival.
The emergence of associative learning has been proposed as one of the key biological innovations that powered the Cambrian explosion by driving the evolution of new sensory modalities and hence, altering the life and adaptive possibilities of animals 37 , 38 . Our results now show that associative learning is also an essential component of plant behaviour. We propose that the ability to construct, remember and recall new relationships established via associative learning constitutes a universal adaptive mechanism shared by all organisms. The ubiquity of associative learning across taxa, including non-animal groups suggests that the role this learning process plays in nature is thus far underexplored and underappreciated. Our findings raise the possibility that associative learning may have played a similarly important role in the remarkable diversification of the plant kingdom and that this kind of learning emerged in plant and animal groups alike via convergent evolution 39 .
Experiment 1: Germination and growth conditions
All experiments in this study have been performed in the garden pea ( Pisum sativum cv Massey Gem). Pea seedlings were germinated hydroponically in 250 mL round containers kept in the dark in a 5.3 m 2 Controlled Environment Room (CER) at the Plant Growth Facilities at the University of Western Australia. First, seeds were soaked in water for 24 hours and then wrapped with clean, wet paper-towel surrounded by an external layer of aluminium foil. Five seeds per roll were used and seed rolls were placed vertically in the round container, immersed in 50 mL of water (replenished daily) and incubated in the dark inside a growth chamber at a constant mean temperature (20 °C ± 0.05 SEM) and humidity (85% ± 0.35 SEM), simultaneously recorded at hourly intervals (U12-011 Temperature/RH Data Logger, HOBO®, Australia). At ca. 09:00 each morning, progress towards germination was evaluated visually in approx. 5–10 sec per seed roll under the dim light of a LED headlamp (with red plastic filter; ca. 0.8 lux). Seeds were considered to have germinated when the radicle was >5 mm long. Ungerminated seeds were discarded. Upon germination, each seedling was planted in soil (Osmocote® seed raising and cutting mix, Scotts Australia) at a depth of 15 mm in the centre of a small black plastic pot (55 mm diameter by 47 mm in depth), which was then sequentially numbered. The soil had been saturated with water and then allowed to drain freely for about 5–10 min prior to planting. Planting occurred in the morning at ca. 09:00 and in the days following planting, plants were watered as needed at this same time. All numbered seedlings were kept in the growth chamber at the same constant temperature and humidity conditions indicated as above, but artificial illumination by cold white fluorescent light was now delivered inside the chamber from the top (at ca. 50 μmol m −2 s −1 at the soil surface) on a 8-h light:16-h dark photoperiod (09:00–17:00 light period) and for a period of 5 to 8 days (depending on emergence times).
Y-maze experimental design
At 09:00 each morning, immediately after light onset, seedlings were checked visually. We recorded the seedlings that emerged out of the soil and were ready to be transferred into their individual custom-designed Y-maze in the morning of the next day to commence training. Each maze unit consisted of a modified 45-degree Y-shaped PVC pipe (dimensions: 55 mm base diameter by 105 mm total height; bifurcation at 65 mm height from base by 95 mm overall width at the bifurcation point; each arm measured 60 mm diameter by 40 mm arm length) fitting on top of the small black plastic pot ( Fig. 1A ) and secured to a polyurethane foam base. The size of the maze was chosen to allow unrestricted growth inside the maze over a three-day period. Each potted seedling within its maze was allocated to one of the two experimental groups using a simple randomization procedure (i.e. coin flipping; the outcome of each throw was recorded next to a list of consecutive numbers in ascending order, which corresponded to the list of numbered pots where peas were to be planted in upon germination). Subsequently, the plants were kept in complete darkness and at a constant 20 °C ± 0.05 SEM ambient temperature throughout the duration of the study. Special care was taken to not disturb the seedlings during this period, including light exposure or any mechanical stimulation, which prevented us from taking regular measures of the rate of growth.
In classical conditioning, the conditioned stimulus (CS) is initially a neutral stimulus that does not naturally trigger a response from the test subject, while the unconditioned stimulus (US) elicits a natural response. In our experiment, we used blue light as US, and fan as CS. To confirm the well-known tropic response of pea seedlings to blue light 12 and thus, the suitability of blue light as the US for this study, we performed a pilot experiment, where a group of 10 seedlings was trained and tested inside a Y-maze where a blue LED light (ca. 14 μmol m −2 s −1 at a 430–505 nm wavelength) was randomly placed on either the left or right arm of the maze and then kept at this fixed position during the entire three-day training and one-day testing phase (Extended Data Fig. 1A ). Before 09:00 the morning following the end of the training phase, all seedlings were checked under the dim red-filtered light of the headlamp as described above to ensure that they had not yet grown into one or the other arm. They were also checked to ensure they had grown to be ca. 1 cm away from the bifurcation of the maze and thus, had to choose a direction for their continuing growth during the following day of testing. At 09:00 in the morning after the testing day, all seedlings were inspected and their position in the maze recorded. All 10 seedlings directed their growth toward the arm of the maze where the light was last presented. Similarly, the airflow produced by a small plastic DC12v mini 9-blade fan (35 mm diameter by 10 mm in depth; speed: 5000 rpm; noise level: 11–15 dBA) was examined as a possible candidate CS.
To test whether the fan itself was a behaviourally neutral cue to pea seedlings, we performed a further pilot experiment, where a group of 30 seedlings was trained and tested inside a Y-maze where a blue light was positioned on each arm of the maze, while a fan was randomly placed on one arm of the maze and then kept at this fixed position during an entire training and testing phase (Extended Data Fig. 1B ). The presence of the fan had no significant effect on seedlings’ direction of growth when light was presented at both ends of the maze simultaneously (Fisher’s Exact Test, P = 0.796, n = 30).
As described above, all seedlings were inspected at the end of the training phase and their position in the maze was recorded in the morning after the testing day. The exact distance of the seedlings from the bifurcation before the testing day was not recorded. Even though this would have been a desirable measurement to record and include as a co-variable, performing it would have introduced the confounding effects of additional stimuli to our experimental design, which would have changed the entire learning paradigm, and made the results difficult to interpret.
The scoring/recording was carried out by two observers using a blind procedure: one observer would score a seedling’s choice and then read out its number, the other would record the score in the logbook by entering the “choice” next to the number and treatment information regarding that seedling. To avoid any observer bias, the observer who had setup the testing conditions on the previous day always recorded, while the other observer always scored.
Experiment 1: Training and testing conditions
Seedlings (n = 45) were kept inside their individual Y-maze at constant temperature and in darkness throughout the experiment, except for the time of blue light exposure. They were randomly assigned to either the condition where fan and light were presented on the same arm of the maze [F + L group] or the condition where they were on opposite arms [F vs L group] ( Fig. 1Ai,ii ). In both experimental groups, the conditioning protocol used to test the associative learning abilities of seedlings consisted of three training days with three training sessions (starting at 09:00, 12:00 and 15:00 respectively) per day, and a subsequent testing day. We employed a Forward Delay Conditioning paradigm, whereby during each training session, the fan as the CS always preceded the blue light as the US by one hour and overlapped with it for 30 min ( Fig. 1B ). Fan and light were always presented in the same position relative to each other according to their assigned group, [F + L] or [F vs L]. Their position in each maze, however, was re-assigned for each training session to ensure that the direction of the incoming light was unpredictable. In a [F + L] maze, for example, fan and light were positioned on the right arm in one training session and then together moved to the left arm for the next session.
To ensure that each pea was exposed to the blue light in an equal number of ‘right’ and ‘left’ sides prior to testing, all peas were trained according to the following pattern: day 1, L/R/L; day 2, L/R/R; day 3, R/L/L and then tested on the right. Each training day consisted of three 2-h training sessions separated by 1-h intervals, during which all seedlings were kept in darkness and undisturbed in their individual mazes ( Fig. 1Bi ). At the end of the 3-d training phase the seedlings were within ca. 1 cm of the bifurcation of the maze and thus, had to choose a direction for their continuing growth during the following day of testing. At this time, seedlings within each experimental group were assigned to either a test group ( Fig. 1Bii ) or a control group ( Fig. 1Biii ) using a simple randomization procedure as described above. In the control group (CTR[F + L], n = 10; CTR[F vs L], n = 9), the seedlings were kept undisturbed in darkness. The seedlings of the test group (n = 13 per experimental group) were also kept in darkness, but exposed to the fan for three 90-min sessions (starting at 09:00, 12:00 and 15:00 respectively) (Extended Data Fig. 1B ). Throughout the three sessions, the fan was positioned either on the same ([F + L]) or the opposite arm of the maze ([F vs L]), where the pea had been exposed to light during its last training session ( Fig. 1A ). This was done to ensure that a possible conditioned response was not confounded by the innate phototropic response to grow into the side of the maze where the light had been presented on the last training session. At 09:00 the morning after the testing day, we inspected the seedlings and recorded the arm of the maze they had grown into. Once scored, seedlings were transferred from the controlled-environment room, transplanted into larger pots and allowed to grow in a glasshouse environment until ready to be replanted in a community garden.
Experiment 2: Germination and growth conditions
In this experiment, dry seeds were first randomly assigned to one of three experimental groups (Light, Light-Dark and Dark, see below). Seeds were initially soaked in water for 24 hours in 250 mL round containers and kept on heated propagation trays. Subsequently, each tray, equipped with a thermostat set at the treatment-specific temperature cycles (recorded at hourly intervals using a HOBO data-logger), was individually housed inside a dark incubation chamber. All experimental groups were maintained at a 12:12 h cycle of ambient temperature (21 °C ± 0.3 SEM during hours 07:00–19:00 (day) and 17 °C ± 0.02 SEM during hours 19:00–07:00 (night). The three experimental groups differed with respect to the timing of their day-night cycle in the chamber relative to external clock time. Specifically, while the 12-h ‘day’ was scheduled from 07:00–19:00 in the first group (Light), it was shifted by 6 hours in the Light-Dark group (01:00–13:00 day: 13:00–01:00 night) and by 12 hours in the Dark group (19:00–07:00 day: 07:00–19:00 night); see yellow and grey colour shaded areas ( Fig. 3A ).
Following the 24-h soaking, seeds were incubated in paper and allowed to germinate hydroponically (as described above) in the dark chambers at their treatment-specific temperature cycles. As in the first experiment, each germinated seedling was planted in wet soil in a small individually numbered plastic pot to a depth of 15 mm. After planting, seedlings from each experimental group were kept in a growth chamber at their specific light:dark/temperature cycle. During this phase, they also received white light illumination delivered inside the chamber from the top as in the learning experiment, but on a 12-h light:12-h dark photoperiod (see yellow:grey shaded areas, Fig. 3A ) synchronized with their group-specific light:dark temperature cycle. The entrainment lasted 5–8 days. Each morning between 06:45 and 07:15, we recorded the seedlings that emerged out of the soil and were ready to be transferred into their individual Y-maze to start training the subsequent morning. To avoid possible confounding effects of changes in light conditions, seedlings in the Dark group were always checked prior to the end of their ‘day’ at 07:00, while those in the Light group were always checked after 07:00, which marked the beginning of their ‘day’.
Each potted seedling within its maze was transferred to a propagation tray heated according to the treatment-specific temperature cycle and kept in darkness for the remainder of the experiment. The temperature cycle served as the Zeitgeber that was maintained throughout the training and testing periods.
Experiment 2: Training and testing conditions
A total of 83 seedlings (Light, n = 28; Light-Dark, n = 30; Dark, n = 25) were individually trained inside a Y-maze, where the fan [F] and the blue LED light [L] were always positioned together on one of the arms of the maze (as in the [F + L] group of the first experiment). The conditioning protocol was the same as that used in the first experiment. It consisted of three training days of three training sessions per 24 hours, and a subsequent testing day, all delivered between 09:00 and 17:00. Accordingly in this experiment, seedlings received their training and tested sessions at times based on their entrained temperature and photoperiod cycles and corresponding to their “subjective” day (Light group), or were partly (Light-Dark group) or entirely outside their subjective day (Extended Data Fig. 2 ). For example, plants from the Light group were trained and tested at a time of day matching their entrained light period, while plants from the Dark group were trained and tested at a time of day, which corresponded to their entrained dark period.
During each training session, the fan exposure as the CS always preceded by 1 hour and overlapped by 30 min with the delivery of blue light as the US ( Fig. 1Bi ). Fan and light were always presented in the same position relative to each other, but their position in each maze was re-assigned for each training session to ensure the source of the light remained unpredictable. Additionally to ensure that each pea was exposed to the blue light in an equal number of ‘right’ and ‘left’ sides prior to testing, we used a simple randomization procedure (as described above) to assign individual peas to one of two alternative 3-day training schedules. Schedule A always started on the right arm and followed the pattern: day 1, R/R/L; day 2, R/L/L; day 3, R/L/R (and tested on the L). Schedule B always started on the left and followed the pattern: day 1, L/L/R; day 2, L/R/R; day 3, L/R/L (and tested on the R). This approach was applied to all peas across experimental groups. As in the first experiment, each training day consisted of three 2-h training sessions separated by 1-h intervals, during which all seedlings were kept in darkness and undisturbed in their individual mazes. During the training phase, seedlings grew and approached the Y-bifurcation of the maze. As described above, all seedlings were checked at the end of the training phase to ensure that they had not yet grown into one or the other arm and had grown to be ca. 1 cm away from the bifurcation of the maze.
At the end of the training phase, seedlings within each group were further split into an experimental sub-group ( Fig. 1Bii ) and a control sub-group ( Fig. 1Biii ). Control seedlings (Light, n = 10; Light-Dark, n = 13; Dark, n = 10) were left in darkness and undisturbed during the testing day. On the testing day, experimental subgroups of seedlings (Light, n = 18; Light-Dark, n = 17; Dark, n = 15) were kept at their treatment-specific temperature cycles in the dark, while being exposed to the fan blowing for three 90-min sessions separated by a 90-min interval. The fan was positioned on the opposite arm of the maze where each pea experienced the light (and the fan) during its last training session. The position of the fan was then maintained fixed for all testing sessions. The morning after the testing day, we inspected all seedlings and recorded which arm of the maze they grew into, scoring whether they made the correct (i.e. towards the fan) or incorrect choice (i.e. away from the fan). Once scored, seedlings were transferred from the controlled-environment room, transplanted into larger pots and later replanted in a community garden.
Phase of the circadian rhythm
The seedlings were initially entrained by a light-dark cycle in conjunction with a temperature cycle. The latter was maintained as sole Zeitgeber during the training and testing phase. Temperature cycle has been previously shown to effectively synchronize circadian rhythms by inducing circadian-type oscillations in the level of RNAs in pea seedlings 16 . The application of three one-hour light pulses during the training days at different phases of the circadian cycle may have induced phase shifts 40 , 41 . Therefore, the phase of the circadian rhythm may have somewhat deviated from the Zeitgeber cycle.
Statistics were based on comparisons between experimental groups (exposure to fan) and control groups (no fan). Since the behaviour of the controls of Experiment 1 and of the ‘Light Group’ of Experiment 2 was determined by last light exposure (phototropism), the question was whether the fan-exposed experimental group could counteract the phototropic effect. The two-tailed Fisher’s Exact Test of independence was used to assess significance.
Additional Information
How to cite this article : Gagliano, M. et al . Learning by Association in Plants. Sci. Rep. 6 , 38427; doi: 10.1038/srep38427 (2016).
Publisher's note: Springer Nature remains neutral with regard to jurisdictional claims in published maps and institutional affiliations.
Merkle, J. A., Fortin, D. & Morales, J. M. A memory-based foraging tactic reveals an adaptive mechanism for restricted space use. Ecol. Lett. 17, 924–931 (2014).
Article CAS Google Scholar
Whitfield, M., Köhler, A. & Nicholson, S. W. Sunbirds increase foraging success by using color as a cue for nectar quality. Behav. Ecol. 25, 328–334 (2014).
Article Google Scholar
Raine, N. E. & Chittka, L. No trade-off between learning speed and associative flexibility in bumblebees: a reversal learning test with multiple colonies. PLoS ONE 7, e45096 (2012).
Article CAS ADS Google Scholar
Clark, J. J., Hollon, N. G. & Phillips, P. E. M. Pavlovian valuation systems in learning and decision making. Curr. Opin. Neurobiol. 22, 1054–1061 (2012).
Aristotle, Nicomachean Ethics: Translation, introduction, and commentary (Trans. and Intro Broadie, S. & Rowe, C. ) (Oxford University Press, 2002).
Stahlman, W., David, W., Young, M. E. & Blaisdell, A. P. Response variability in pigeons in a Pavlovian task. Learn. Mem. 38, 111–118 (2011).
Google Scholar
Nilsson, J., Kristiansen, T. S., Fosseidengen, J. E., Fernö, A. & Bos, R. Sign- and goal-tracking in Atlantic cod ( Gadus morhua ). Anim. Cogn. 11, 651–659 (2008).
Gil, M., De Marco, R. J. & Menzel, R. Learning reward expectations in honeybees. Learn. Mem. 14, 491–496 (2007).
Watanabe, M. et al. Behavioral reactions reflecting differential reward expectations in monkeys. Exp. Brain Res. 140, 511–518 (2001).
Graf, A. & Smith, A. M. Starch and the clock: the dark side of plant productivity. Trends Plant Sci. 16, 169–175 (2011).
Gagliano, M., Renton, M., Depczynski, M. & Mancuso, S. Experience teaches plants to learn faster and forget slower in environments where it matters. Oecologia 175, 63–72 (2014).
Article ADS Google Scholar
Christie, J. M. Phototropin blue-light receptors. Annu. Rev. Plant Biol. 58, 21–45 (2007).
Dibner, C., Schibler, U. & Albrecht, U. The mammalian circadian timing system: organization and coordination of central and peripheral clocks. Annu. Rev. Physiol. 72, 517–549 (2010).
Krishnan, H. C. & Lyons, L. C. Synchrony and desynchrony in circadian clocks: impacts on learning and memory. Learn Mem. 22, 426–437 (2015).
Robertson McClung, C. Plant circadian rhythms. Plant Cell 18, 792–803 (2006).
Kloppstech, K., Otto, B. & Sierralta, W. Cyclic temperature treatments of dark-grown pea seedlings induce a rise in specific transcript levels of light-regulated genes related to photomorphogenesis. Mol. Gen. Genet. 225, 468–473 (1991).
Molet, M. & Miller, R. R. Timing: an attribute of associative learning. Behav. Process. 101, 4–14 (2014).
Shettleworth, S. J. Animal behaviour: planning for breakfast. Nature 445, 825–826 (2007).
Heyes, C. Simple minds: a qualified defence of associative learning. Phil. Trans. R. Soc. B 367, 2695–2703 (2012).
Goldschmidt, D., Wörgötter, F. & Manoonpong, P. Biologically-inspired adaptive obstacle negotiation behavior of hexapod robots. Front. Neurorobot. 8, 3 (2014).
Armus, H. L. “Conditioning of the sensitive plant, Mimosa pudica ,” In Comparative Psychology: Research in Animal Behavior (eds Denny, M. R. & Ratner, S. C. ) 597–600 (Dorsey Press, 1970).
Abramson, C. I. et al. Bioelectrical potentials of Philodendron cordatum : A new method for the investigation of behavior in plants. Psychol. Rep. 91, 173–185 (2002).
Urcelay, G. P. & Miller, R. R. The functions of contexts in associative learning. Behav. Proc. 104, 2–12 (2014).
de Mendonça-Furtado, O. & Ottoni, E. B. Learning generalization in problem solving by a blue-fronted parrot ( Amazona aestiva ). Anim. Cogn. 11, 719–725 (2008).
Wismer, S., Grutter, A. & Bshary, R. Generalized rule application in bluestreak cleaner wrasse ( Labroides dimidiatus ): using predator species as social tools to reduce punishment. Anim. Cogn. 19, 769–778 (2016).
Ginsburg, S. & Jablonka, E. Epigenetic learning in non-neural organisms; J. Biosci. 34, 633–646 (2009).
Ledón-Rettig, C. C., Richards, C. L. & Martin, L. B. Epigenetics for behavioral ecologists. Behav. Ecol. 24, 311–324 (2013).
Thellier, M. & Lüttge, U. Plant memory: a tentative model. Plant. Biol. 15, 1–12 (2013).
Bray, D. Molecular networks: the top-down view. Science 301, 1864–1865 (2003).
Rankin, C. H. A bite to remember. Science 296, 164–165 (2002).
Carew, T. J. Understanding the consequences. Nature 417, 803–806 (2002).
Telewski, F. W. A unified hypothesis of mechanoreception in plants. Am. J. Bot. 93, 1466–1476 (2006).
Halliday, K. J., Martínez-García, J. F. & Josse, E.-M. Integration of light and auxin signalling. Cold Spring Harb. Perspect. Biol. 1, a001586 (2009).
Nick, P. & Schafer, E. Spatial memory during the tropism of maize ( Zea mays L.) coleoptiles. Planta 175, 380–388 (1988).
de Montaigu, A., Toth, R. & Coupland, G. Plant development goes like clockwork. Trends Genet. 26, 296–306 (2010).
Graf, A., Schlereth, A., Stitt, M. & Smith, A. M. Circadian control of carbohydrate availability for growth in Arabidopsis plants at night. Proc. Natl. Acad. Sci. USA 107, 9458–9463 (2010).
Ginsburg, S. & Jablonka, E. The evolution of associative learning: a factor in the Cambrian explosion. J. Theor. Biol. 266, 11–20 (2010).
Conway Morris, S. The Cambrian “explosion”: Slow-fuse or megatonnage? Proc. Natl. Acad. Sci. USA 97, 4426–4429 (2000).
Conway Morris, S. Evolutionary convergence. Curr. Biol. 16, R826–R827 (2006).
Covington, F. et al. ELF3 modulates resetting of the circadian clock in Arabidopsis . Plant Cell 13, 1305–1315 (2001).
Ohara, T., Fukuda, H. & Tokuda, I. T. Phase response of the Arabidopsis thaliana circadian clock to light pulses of different wavelength. J. Biol. Rhythms 30, 95–103 (2015).
Download references
Acknowledgements
We thank C. Cirelli and J. Tomkins for early discussions on aspects of the study, C. Silvano, R. Creasy, W. Piasini and P. Tallai for technical assistance, B. Radford for statistical advice, L. Simmons for commenting on an earlier draft and S. Whalan and 3 anonymous reviewers for their constructive feedback. This research was supported by grants from the University of Western Australia and the Australian Research Council to M. Gagliano.
Author information
Authors and affiliations.
Centre for Evolutionary Biology, School of Animal Biology, University of Western Australia, Crawley, 6009, WA, Australia
Monica Gagliano & Mavra Grimonprez
Department of Physiology, Anatomy and Genetics, University of Oxford, Oxford, OX1 3PT, United Kingdom
Vladyslav V. Vyazovskiy
Institute of Pharmacology and Toxicology, University of Zurich, Zurich, 8057, Switzerland
Alexander A. Borbély
Australian Institute of Marine Science, Crawley, 6009, WA, Australia
Martial Depczynski
Oceans Institute, University of Western Australia, Crawley, WA, Australia
You can also search for this author in PubMed Google Scholar
Contributions
M.G.* implemented the analysis and drafted the results; M.G.* and M.D.* planned the experiments, which were conducted by M.G.* and M.G.; M.G.*, V.V.V., A.A.B. and M.D. wrote the paper.
Ethics declarations
Competing interests.
The authors declare no competing financial interests.
Electronic supplementary material
Supplementary information, supplementary video 1, supplementary dataset 1, rights and permissions.
This work is licensed under a Creative Commons Attribution 4.0 International License. The images or other third party material in this article are included in the article’s Creative Commons license, unless indicated otherwise in the credit line; if the material is not included under the Creative Commons license, users will need to obtain permission from the license holder to reproduce the material. To view a copy of this license, visit http://creativecommons.org/licenses/by/4.0/
Reprints and permissions
About this article
Cite this article.
Gagliano, M., Vyazovskiy, V., Borbély, A. et al. Learning by Association in Plants. Sci Rep 6 , 38427 (2016). https://doi.org/10.1038/srep38427
Download citation
Received : 26 July 2016
Accepted : 08 November 2016
Published : 02 December 2016
DOI : https://doi.org/10.1038/srep38427
Share this article
Anyone you share the following link with will be able to read this content:
Sorry, a shareable link is not currently available for this article.
Provided by the Springer Nature SharedIt content-sharing initiative
This article is cited by
Science fosters ongoing reassessments of plant capabilities.
- Hyrandir Cabral de Melo
Theoretical and Experimental Plant Physiology (2024)
Evaluation of secondary sexual dimorphism of the dioecious Amaranthus palmeri under abiotic stress
- Nicholas E. Korres
- Jason K. Norsworthy
- Govindjee Govindjee
Scientific Reports (2023)
What is cognitive about ‘plant cognition’?
Biology & Philosophy (2023)
Many Paths to Anticipatory Behavior: Anticipatory Model Acquisition Across Phylogenetic and Ontogenetic Timescales
- Matthew Sims
Biological Theory (2023)
Rethinking economic theories of plant water use
- Adam B. Roddy
Journal of Biosciences (2023)
By submitting a comment you agree to abide by our Terms and Community Guidelines . If you find something abusive or that does not comply with our terms or guidelines please flag it as inappropriate.
Quick links
- Explore articles by subject
- Guide to authors
- Editorial policies
Sign up for the Nature Briefing newsletter — what matters in science, free to your inbox daily.

- Coordinator Login
- Teacher Login
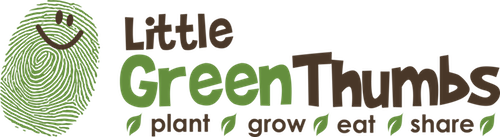
Experiment: Plant Light Shoebox Maze Experiment
Grades: K – 7
An experiment to test if a plant can find its way through a box maze towards light.
Set up: 45 minutes Growing time: 3-4 days, depending on seed variety
The word phototropism is a mouthful! It’s made up of two parts: photo which means light and tropism which comes from the Greek word tropos meaning to turn.
To grow tall and strong, plants use energy from light to make food in a process called photosynthesis. We think of plants as being stuck in one place, but they can move their stems to grow towards light. This movement is called phototropism: where plants turn or orient towards a source of light. This happens in plants and even some fungi.
Here’s how it works: Plants use many hormones to grow. One hormone in particular called auxin, tells individual cells to grow longer. It’s one of the ways that plants grow taller. Normally, plants growing with an unshaded light source will grow straight up towards the sun because auxin is evenly distributed all around the shoot.
But when something blocks or shades the sun like a tree or other plants, something interesting happens. Auxin starts to concentrate on the shaded side of the plant instead, and as a result, the cells on the sunny side stay the same size but the cells on the shaded side grow longer. This causes the plant to turn or bend towards the light.
Phototropism is an evolutionary adaptation that helps plants to move access light needed for photosynthesis.
Materials needed for the plant:
- Small plastic plant container about 7 – 10 cm high (or recycled paper cups with a drainage hole in the bottom) Plant Light Shoebox Maze Experiment
- Potting soil (soilless medium)Cotton balls or rockwool
- 2 – 3 pole bean seeds per plant container (ensure that you use pole bean seeds which grow long and tall, not bush beans which are short)
Materials needed for the shoebox maze:
- Shoebox with lid
- Scraps of light cardboard
- Duct tape or masking tape
- Science journal to record observations
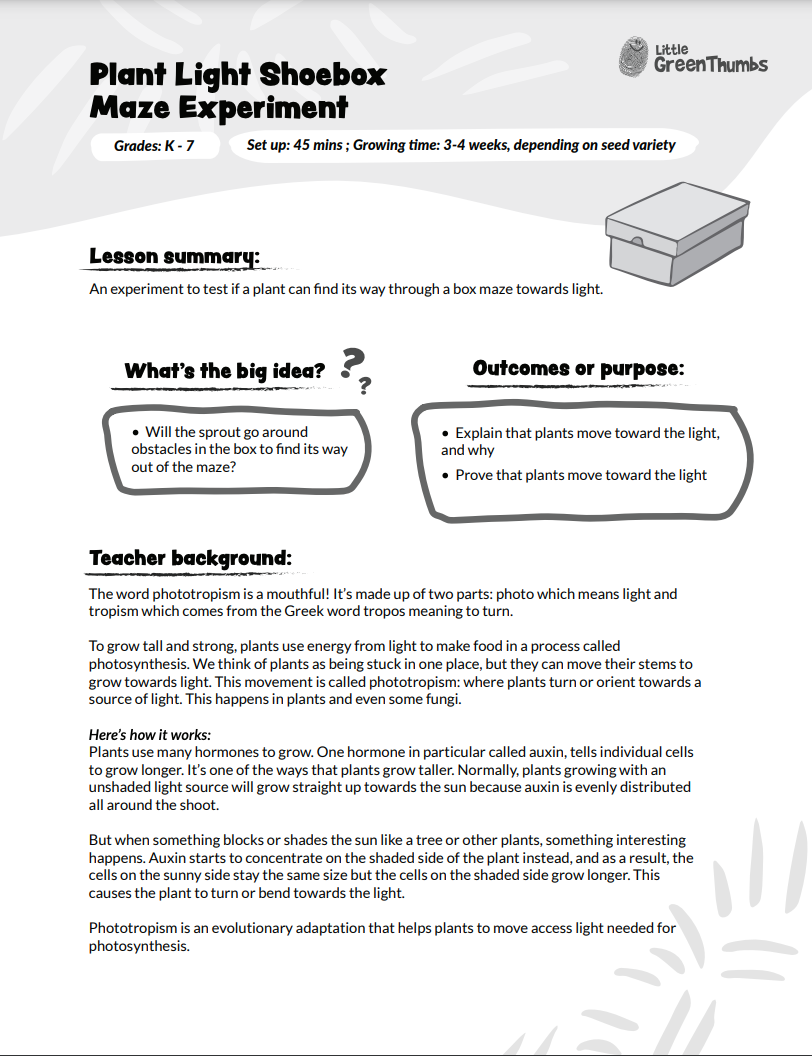
Discussion questions and activities
How long did the plant take to grow through the maze?
What things do you think help a plant grow faster or slower?
Why do plants grow towards where the light is? How does light help a plant grow? What else is light useful for?
What makes a shadow? Why are shadows important for plants, animals, and people? Do you think plants like or don't like shadows? Why?
How tall is your plant? What other things are this size? Can you name three things that are bigger than your plant and three things that are smaller than your plant?
Did you know that you can tell how old a tree is by the rings in its trunk? If a tree trunk is cut, you can see rings inside. Each ring is one year of the tree's life. So, if you count all the rings, you will know how many years old the tree is. For example, if there are ten rings, the tree is ten years old.
Many trees live longer than animals and even dinosaurs. Research how long trees can live and how long different animals live. What animals live the longest? What trees live the longest?
Have you enjoyed learning about light, nature, and time? Keep exploring at our interactive installation Superluminal , showing at the South Australian Museum, in July/August 2024.
More activities to try
-1668046389.png)
New insights into the response of maize to fluctuations in the light environment
- Original Article
- Published: 25 February 2021
- Volume 296 , pages 615–629, ( 2021 )

Cite this article
- Qu Jianzhou 1 , 2 ,
- Xiaonan Gou 1 , 2 ,
- Wenxin Zhang 1 , 2 ,
- Ting Li 1 , 2 ,
- Jiquan Xue 1 , 2 ,
- Dongwei Guo 1 , 2 &
- Shutu Xu ORCID: orcid.org/0000-0003-4153-6373 1 , 2
718 Accesses
3 Citations
Explore all metrics
Light is the most important environmental cue signaling the transition from skotomorphogenesis to photomorphogenesis, thus affecting plant development and metabolic activity. How the light response mechanisms of maize seedlings respond to fluctuations in the light environment has not been well characterized to date. In this study, we built a gene coexpression network from a dynamic transcriptomic map of maize seedlings exposed to different light environments. Coexpression analysis identified ten modules and multiple genes that closely correlate with photosynthesis and characterized hub genes associated with regulatory networks, duplication events, domestication and improvement. In addition, we identified that 38% of hub genes underwent duplication events, 74% of which are related to photosynthesis. Moreover, we captured the dynamic expression atlas of gene sets involved in the chloroplast photosynthetic apparatus and photosynthetic carbon assimilation in different light environments, which should help to elucidate the key mechanisms and regulatory networks that underlie photosynthesis in maize. Insights from this study provide a valuable resource to better understand the genetic mechanisms of the response to fluctuations in the light environment in maize.
This is a preview of subscription content, log in via an institution to check access.
Access this article
Price includes VAT (Russian Federation)
Instant access to the full article PDF.
Rent this article via DeepDyve
Institutional subscriptions
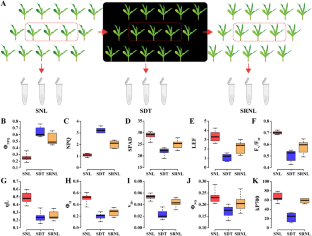
Similar content being viewed by others
Regulatory modules controlling early shade avoidance response in maize seedlings.
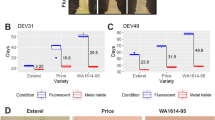
Diversity of gene expression responses to light quality in barley
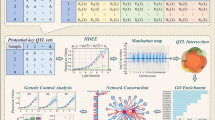
Computational dissection of genetic variation modulating the response of multiple photosynthetic phenotypes to the light environment
Data availability.
Sequence data from this article can be found in the National Center for Biotechnology Information Sequence Read Archive ( http://www.ncbi.nlm.nih.gov/sra ) under accession number SRP118761.
Achard P, Liao L, Jiang C, Desnos T, Bartlett J, Fu X, Harberd NP (2007) DELLAs contribute to plant photomorphogenesis. Plant Physiol 143:1163–1172
CAS PubMed PubMed Central Google Scholar
Allen JF (2002) Photosynthesis of ATP—electrons, proton pumps, rotors, and poise. Cell 110:273–276
CAS PubMed Google Scholar
Alter P, Dreissen A, Luo FL, Matsubara S (2012) Acclimatory responses of Arabidopsis to fluctuating light environment: comparison of different sunfleck regimes and accessions. Photosynth Res 113:221–237
Assenov Y, Ramirez F, Schelhorn SE, Lengauer T, Albrecht M (2008) Computing topological parameters of biological networks. Bioinformatics 24:282–284
Ballesteros ML, Bolle C, Lois LM, Moore JM, Vielle-Calzada JP, Grossniklaus U, Chua NH (2001) LAF1, a MYB transcription activator for phytochrome A signaling. Genes Dev 15:2613–2625
Baumgardt RL, Oliverio KA, Casal JJ, Hoecker U (2002) SPA1, a component of phytochrome A signal transduction, regulates the light signaling current. Planta 215:745–753
Benjamini Y, Hochberg Y (1995) Controlling the false discovery rate: a practical and powerful approach to multiple testing. J Roy Statist Soc Ser B 57:289–300
Google Scholar
Boeckx T, Winters AL, Webb KJ, Kingston-Smith AH (2015) Polyphenol oxidase in leaves: is there any significance to the chloroplastic localization? J Exp Bot 66:3571–3579
Brohammer AB, Kono TJY, Springer NM, McGaugh SE, Hirsch CN (2018) The limited role of differential fractionation in genome content variation and function in maize (Zea mays L.) inbred lines. Plant J 93:131–141
Buchanan BB, Balmer Y (2005) Redox regulation: a broadening horizon. Annu Rev Plant Biol 56:187–220
Chattopadhyay S, Ang LH, Puente P, Deng XW, Wei N (1998) Arabidopsis bZIP protein HY5 directly interacts with light-responsive promoters in mediating light control of gene expression. Plant Cell 10:673–683
Cockburn W (1983) C3 C4: Mechanisms, and cellular and environmental regulation, of photosynthesis (Book). Plant Cell Environ 6:747–748
Darko E, Heydarizadeh P, Schoefs B, Sabzalian MR (2014) Photosynthesis under artificial light: the shift in primary and secondary metabolism. Philos Trans R Soc Lond B Biol Sci 369:20130243
PubMed PubMed Central Google Scholar
de Wit M, Galvao VC, Fankhauser C (2016) Light-mediated hormonal regulation of plant growth and development. Annu Rev Plant Biol 67:513–537
PubMed Google Scholar
Deng X-W, Matsui M, Wei N, Wagner D, Chu AM, Feldmann KA, Quail PH (1992) COP1, an Arabidopsis regulatory gene, encodes a protein with both a zinc-binding motif and a G beta homologous domain. Cell 71:791–801
Deng XW, Matsui M, Wei N, Wagner D, Chu AM, Feldmann KA, Quail PH (1994) Light inactivation of arabidopsis photomorphogenic repressor COP1 involves a cell-specific regulation of its ucleocytoplasmic partitioning. Cell 79:1035–1045
Du Z, Zhou X, Ling Y, Zhang Z, Su Z (2010) agriGO: a GO analysis toolkit for the agricultural community. Nucleic Acids Res 38:W64-70
Eberhard S, Finazzi G, Wollman FA (2008) The dynamics of photosynthesis. Annu Rev Genet 42:463–515
Fitter DW, Martin DJ, Copley MJ, Scotland RW, Langdale JA (2002) GLK gene pairs regulate chloroplast development in diverse plant species. Plant J 31:713–727
Fleet CM, Sun TP (2005) A DELLAcate balance: the role of gibberellin in plant morphogenesis. Curr Opin Plant Biol 8:77–85
Freeling M (2009) Bias in plant gene content following different sorts of duplication: tandem, whole-genome, segmental, or by transposition. Annu Rev Plant Biol 60:433–453
Garrone A, Archipowa N, Zipfel PF, Hermann G, Dietzek B (2015) Plant protochlorophyllide oxidoreductases A and B: catalytic efficiency and initial reaction steps. J Biol Chem 290:28530–28539
Giuliani R, Karki S, Covshoff S, Lin HC, Coe RA, Koteyeva NK, Evans MA, Quick WP, von Caemmerer S, Furbank RT, Hibberd JM, Edwards GE, Cousins AB (2019) Transgenic maize phosphoenolpyruvate carboxylase alters leaf-atmosphere CO2 and (13)CO2 exchanges in Oryza sativa. Photosynth Res. https://doi.org/10.1007/s11120-019-00655-4
Article PubMed PubMed Central Google Scholar
Grossman AR, Bhaya D, Apt KE, Kehoe DM (1995) LIGHT-harvesting complexes in oxygenic photosynthesis: diversity, control, and evolution. Ann Rev Genet 29:231–288
Hanada K, Kuromori T, Myouga F, Toyoda T, Li WH, Shinozaki K (2009) Evolutionary persistence of functional compensation by duplicate genes in Arabidopsis. Genome Biol Evol 1:409–414
He JX, Gendron JM, Sun Y, Gampala SS, Gendron N, Sun CQ, Wang ZY (2005) BZR1 is a transcriptional repressor with dual roles in brassinosteroid homeostasis and growth responses. Science 307:1634–1638
Hornitschek P, Kohnen MV, Lorrain S, Rougemont J, Ljung K, Lopez-Vidriero I, Franco-Zorrilla JM, Solano R, Trevisan M, Pradervand S, Xenarios I, Fankhauser C (2012) Phytochrome interacting factors 4 and 5 control seedling growth in changing light conditions by directly controlling auxin signaling. Plant J 71:699–711
Hufford MB, Xu X, van Heerwaarden J, Pyhajarvi T, Chia JM, Cartwright RA, Elshire RJ, Glaubitz JC, Guill KE, Kaeppler SM, Lai J, Morrell PL, Shannon LM, Song C, Springer NM, Swanson-Wagner RA, Tiffin P, Wang J, Zhang G, Doebley J, McMullen MD, Ware D, Buckler ES, Yang S, Ross-Ibarra J (2012) Comparative population genomics of maize domestication and improvement. Nat Genet 44:808–811
Jensen RG (1983) Photosynthesis: C3, C4. mechanisms, and cellular and environmental regulation, of photosynthesis. Science 222:1009–1009
Jin J, Tian F, Yang DC, Meng YQ, Kong L, Luo J, Gao G (2017) PlantTFDB 4.0: toward a central hub for transcription factors and regulatory interactions in plants. Nucleic Acids Res 45:D1040–D1045
Kanehisa M, Furumichi M, Tanabe M, Sato Y, Morishima K (2017) KEGG: new perspectives on genomes, pathways, diseases and drugs. Nucleic Acids Res 45:D353–D361
Kaur N, Li J, Hu J (2013) Peroxisomes and Photomorphogenesis. In: del Río LA (ed) Peroxisomes and their Key Role in Cellular Signaling and Metabolism. Springer, Netherlands, Dordrecht, pp 195–211
Kim B, Jeong YJ, Corvalan C, Fujioka S, Cho S, Park T, Choe S (2014) Darkness and gulliver2/phyB mutation decrease the abundance of phosphorylated BZR1 to activate brassinosteroid signaling in Arabidopsis. Plant J 77:737–747
Kramer DM, Avenson TJ, Edwards GE (2004) Dynamic flexibility in the light reactions of photosynthesis governed by both electron and proton transfer reactions. Trends Plant Sci 9:349–357
Kuhlgert S, Austic G, Zegarac R, Osei-Bonsu I, Hoh D, Chilvers MI, Roth MG, Bi K, TerAvest D, Weebadde P, Kramer DM (2016) MultispeQ Beta: a tool for large-scale plant phenotyping connected to the open PhotosynQ network. R Soc Open Sci 3:160592
Langfelder P, Horvath S (2008) WGCNA: an R package for weighted correlation network analysis. BMC Bioinformatics 9:559
Langmead B, Trapnell C, Pop M, Salzberg SL (2009) Ultrafast and memory-efficient alignment of short DNA sequences to the human genome. Genome Biol 10:R25
Li T, Jia KP, Lian HL, Yang X, Li L, Yang HQ (2014) Jasmonic acid enhancement of anthocyanin accumulation is dependent on phytochrome A signaling pathway under far-red light in Arabidopsis. Biochem Biophys Res Commun 454:78–83
Li QF, Huang LC, Wei K, Yu JW, Zhang CQ, Liu QQ (2017) Light involved regulation of BZR1 stability and phosphorylation status to coordinate plant growth in Arabidopsis. Biosci Rep. https://doi.org/10.1042/BSR20170069
Lu M, Yang G, Li P, Wang Z, Fu S, Zhang X, Chen X, Shi M, Ming Z, Xia J (2018) Bioinformatic and functional analysis of a key determinant underlying the substrate selectivity of the Al transporter, Nrat1. Front Plant Sci 9:606
Luo XM, Lin WH, Zhu S, Zhu JY, Sun Y, Fan XY, Cheng M, Hao Y, Oh E, Tian M, Liu L, Zhang M, Xie Q, Chong K, Wang ZY (2010) Integration of light- and brassinosteroid-signaling pathways by a GATA transcription factor in Arabidopsis. Dev Cell 19:872–883
Menon C, Sheerin DJ, Hiltbrunner A (2016) SPA proteins: SPAnning the gap between visible light and gene expression. Planta 244:297–312
Miao Z, Han Z, Zhang T, Chen S, Ma C (2017) A systems approach to a spatio-temporal understanding of the drought stress response in maize. Sci Rep 7:6590
Murchie EH, Niyogi KK (2011) Manipulation of photoprotection to improve plant photosynthesis. Plant Physiol 155:86–92
Okada K (2009) PetH is rate-controlling in the interaction between PetH, a component of the supramolecular complex with photosystem II, and PetF, a light-dependent electron transfer protein. Biochem Biophys Res Commun 389:394–398
Oyama T, Shimura Y, Okada K (1997) The Arabidopsis HY5 gene encodes a bZIP protein that regulates stimulus-induced development of root and hypocotyl. Genes Dev 11:2983–2995
Pahari S, Cormark RD, Blackshaw MT, Liu C, Erickson JL, Schultz EA (2014) Arabidopsis UNHINGED encodes a VPS51 homolog and reveals a role for the GARP complex in leaf shape and vein patterning. Development 141:1894–1905
Panchy N, Lehti-Shiu M, Shiu SH (2016) Evolution of gene duplication in plants. Plant Physiol 171:2294–2316
Parks BM (2003) The red side of photomorphogenesis. Plant Physiol 133:1437–1444
Ponnu J, Wahl V, Schmid M (2011) Trehalose-6-phosphate: connecting plant metabolism and development. Front Plant Sci 2:70
Robinson MD, McCarthy DJ, Smyth GK (2010) edgeR: a Bioconductor package for differential expression analysis of digital gene expression data. Bioinformatics 26:139–140
Sakata S, Mizusawa N, Kubota-Kawai H, Sakurai I, Wada H (2013) Psb28 is involved in recovery of photosystem II at high temperature in Synechocystis sp. PCC 6803. Biochim Biophys Acta 1827:50–59
Scharf KD, Berberich T, Ebersberger I, Nover L (2012) The plant heat stress transcription factor (Hsf) family: structure, function and evolution. Biochim Biophys Acta 1819:104–119
Schnable JC, Springer NM, Freeling M (2011) Differentiation of the maize subgenomes by genome dominance and both ancient and ongoing gene loss. Proc Natl Acad Sci USA 108:4069–4074
Schottler MA, Toth SZ (2014) Photosynthetic complex stoichiometry dynamics in higher plants: environmental acclimation and photosynthetic flux control. Front Plant Sci 5:188
Schottler MA, Toth SZ, Boulouis A, Kahlau S (2015) Photosynthetic complex stoichiometry dynamics in higher plants: biogenesis, function, and turnover of ATP synthase and the cytochrome b6f complex. J Exp Bot 66:2373–2400
Schumann T, Paul S, Melzer M, Dormann P, Jahns P (2017) Plant growth under natural light conditions provides highly flexible short-term acclimation properties toward high light stress. Front Plant Sci 8:681
Shannon P, Markiel A, Ozier O, Baliga NS, Wang JT, Ramage D, Amin N, Schwikowski B, Ideker T (2003) Cytoscape: a software environment for integrated models of biomolecular interaction networks. Genome Res 13:2498–2504
Shi Q, Kong F, Zhang H, Ye J, Heng S, Liang R, Ma L, Liu J, Lu X, Li P, Li G (2019) Molecular mechanisms governing shade responses in maize. Biochem Biophys Res Commun 516:112–119
Shikanai T (2016) Chloroplast NDH: A different enzyme with a structure similar to that of respiratory NADH dehydrogenase. Biochim Biophys Acta 1857:1015–1022
Smith AM, Zeeman SC, Smith SM (2005) Starch degradation. Annu Rev Plant Biol 56:73–98
Trapnell C, Roberts A, Goff L, Pertea G, Kim D, Kelley DR, Pimentel H, Salzberg SL, Rinn JL, Pachter L (2012) Differential gene and transcript expression analysis of RNA-seq experiments with TopHat and Cufflinks. Nat Protoc 7:562–578
Wang H, Wu G, Zhao B, Wang B, Lang Z, Zhang C, Wang H (2016) Regulatory modules controlling early shade avoidance response in maize seedlings. BMC Genomics 17:269
Wang B, Lin Z, Li X, Zhao Y, Zhao B, Wu G, Ma X, Wang H, Xie Y, Li Q, Song G, Kong D, Zheng Z, Wei H, Shen R, Wu H, Chen C, Meng Z, Wang T, Li Y, Li X, Chen Y, Lai J, Hufford MB, Ross-Ibarra J, He H, Wang H (2020) Genome-wide selection and genetic improvement during modern maize breeding. Nat Genet. https://doi.org/10.1038/s41588-020-0616-3
Weaver LM, Amasino RM (2001) Senescence is induced in individually darkened arabidopsis leaves, but inhibited in whole darkened plants. Plant Physiol 127:876–886
Weise SE, Weber AP, Sharkey TD (2004) Maltose is the major form of carbon exported from the chloroplast at night. Planta 218:474–482
Wise RR, Hoober JK (2006) The Diversity of Plastid Form and Function. Springer, The Netherlands, pp 3–26
Wu SH (2014) Gene expression regulation in photomorphogenesis from the perspective of the central dogma. Annu Rev Plant Biol 65:311–333
Yilmaz A, Nishiyama MY Jr, Fuentes BG, Souza GM, Janies D, Gray J, Grotewold E (2009) GRASSIUS: a platform for comparative regulatory genomics across the grasses. Plant Physiol 149:171–180
Zhang J (2012) Genetic Redundancies and Their Evolutionary Maintenance. In: Soyer OS (ed) Evolutionary Systems Biology. Springer, New York , pp 279–300
Download references
Acknowledgements
This study was funded by the Young Scientists Fund of the National Natural Science Foundation of China (Grant No. 31701438) and the Science Foundation for Post Doctorate Research of the Ministry of Science and Technology of China (2018M633588).
Author information
Authors and affiliations.
The Key Laboratory of Biology and Genetic Improvement of Maize in Arid Area of Northwest Region, College of Agronomy, Northwest A&F University, Yangling, 712100, Shaanxi, China
Qu Jianzhou, Xiaonan Gou, Wenxin Zhang, Ting Li, Jiquan Xue, Dongwei Guo & Shutu Xu
Maize Engineering Technology Research Centre of Shaanxi Province, Yangling, 712100, Shaanxi, China
You can also search for this author in PubMed Google Scholar
Contributions
Shutu Xu, Dongwei Guo and Jiquan Xue conceived and designed the experiments. Shutu Xu, Xiaonan Gou, Wenxin Zhang, Ting Li and Jianzhou Qu performed experiments. Jianzhou Qu analyzed and interpreted the data. Jianzhou Qu and Shutu Xu wrote the paper and prepared figures and/or tables. All authors read and approved the final manuscript.
Corresponding author
Correspondence to Jiquan Xue .
Ethics declarations
Conflicts of interest.
All the authors declare that they have no conflicts of interest.
Ethical approval
This article does not contain any studies with human participants or animals performed by any of the authors.
Additional information
Communicated by Stefan Hohmann.
Publisher's Note
Springer Nature remains neutral with regard to jurisdictional claims in published maps and institutional affiliations.
Supplementary Information
Below is the link to the electronic supplementary material.
438_2021_1761_MOESM1_ESM.tif
Supplementary Correlation of gene expression level across replicates. For each gene, the expression values were normalized by the log2(FPKM+1). The scale bar shows the degree of correlation between samples. The samples from seedlings under normal light condition (SNL), seedlings after four days of dark treatment (SDT) and four days after seedlings return to normal light (SRNL) (TIF 2312 KB)
438_2021_1761_MOESM2_ESM.tif
Supplementary Comparison of expression patterns of candidate genes by qRT-PCR and RNA-Seq. Histogram showing expression levels of candidate genes based on qRT-PCR (left). Boxplot showing expression levels of candidate genes based on RNA-Seq (right). The samples from seedlings under normal light condition (SNL), seedlings after four days of dark treatment (SDT) and four days after seedlings return to normal light (SRNL) (TIF 3827 KB)
Supplementary file3 (XLSX 11 KB)
Supplementary file4 (xlsx 10 kb), supplementary file5 (xlsx 3903 kb), supplementary file6 (xlsx 4165 kb), supplementary file7 (xlsx 18 kb), supplementary file8 (xlsx 129 kb), supplementary file9 (xlsx 84 kb), supplementary file10 (xlsx 6208 kb), supplementary file11 (xlsx 67 kb), supplementary file12 (xlsx 110 kb), supplementary file13 (xlsx 8669 kb), supplementary file14 (xlsx 16 kb), supplementary file15 (xlsx 125 kb), rights and permissions.
Reprints and permissions
About this article
Qu, J., Gou, X., Zhang, W. et al. New insights into the response of maize to fluctuations in the light environment. Mol Genet Genomics 296 , 615–629 (2021). https://doi.org/10.1007/s00438-021-01761-6
Download citation
Received : 25 September 2020
Accepted : 11 January 2021
Published : 25 February 2021
Issue Date : May 2021
DOI : https://doi.org/10.1007/s00438-021-01761-6
Share this article
Anyone you share the following link with will be able to read this content:
Sorry, a shareable link is not currently available for this article.
Provided by the Springer Nature SharedIt content-sharing initiative
- Maize seedling
- RNA sequencing
- Photosynthesis
- Find a journal
- Publish with us
- Track your research
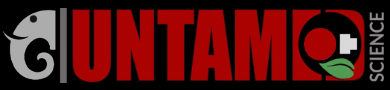
- Plant Biology
- Human Biology
- Biology Plant Biology Phototropism Experiment
Experiment: How do plants “see” light?
Can plants really “see” the light.
Scientists call a plant’s ability to bend toward light phototropism . Even as far back as ancient Greece it’s been a big puzzle about how plants are able to do it. People experimented with how plants accomplish this amazing feat, but no one really figured out how it worked—until Charles Darwin came along, that is.
Although Darwin is most well-known for his studies on evolution, he was also a prolific scientist in general. The questions about phototropism piqued his curiosity, and he thought of an ingenious experiment to test how plants are able to see light. In this experiment, we’ll recreate what he did, and at the end we’ll dive further into the science.

3 small cups full of soil Tape, a marker, and 3 sticky notes Medium-sized box (such as a shoebox or a storage cube) 12 corn seeds Aluminum foil Small cookie sheet that fits inside the box (or another sheet of aluminum foil) 1 Straw Water
- Plant four corn seeds in each of the soil cups. Make sure they’re evenly spaced, and plant them just a half inch under the dirt.
- Water the cups, and dump out any excess water (be careful not to tip the soil and seeds out). Place the cups on the cookie sheet or aluminum foil. This will prevent moisture and dirt from soaking through the box.
- Place the cups/cookie sheet setup inside of the box. Make sure it’s open on one side so that light is coming in from an angle. Place in a windowsill, with the open side facing the sun. (You might need to stack some books underneath it to support it, if your windowsill isn’t very wide.)
- Shoot cap: Cut a small 2″ x 3″ square of aluminum foil. Wrap it around the tip of a straw to create a small, closed-ended metal cap, and slide it off. This will be placed over the tip of the growing shoot to cover any light coming in to the tip.
- Base sleeve: Cut a small 1/2″ x 3″ square of aluminum foil. Wrap it around the middle of a straw so it creates a small open-ended 1/2″ tall tube, and slide it off. This will be placed around the growing shoot so that it can grow through it.
- Check the cups each day. Once they send up a shoot about half an inch high, place either a shoot cap (on Tip seedlings) or a base sleeve (on Base seedlings) around them, depending on which cup they’re in. The control cup will get neither of the light exclusion devices. The seedlings might grow at different rates, so be sure to check each day to put the caps/sleeves on as needed. They grow fast once they germinate!
- Continue to water the seedlings as needed.
- Check the seedlings after a week. What has happened? Compare the seedlings with the caps and the sleeves to the control seedlings. Are any of them growing in certain directions?
How did the seedlings “see” the light?
If the experiment worked correctly, you should have noticed that the seedlings that were covered with caps at the tip grew straight up, while the control seedlings and the seedlings with the bases covered bent towards the light. This is phototropism in action.
Darwin correctly concluded that plants are able to “see” light using the tips of the plant shoots, rather than through the stalks. It wasn’t until a bit later that scientists figured out exactly why that was, though.
It turns out that plants are able to grow by using hormones such as auxins and gibberellins . Auxin in particular tells individual cells to reach out and grow longer, like Stretch Armstrong. It’s one of the ways that plants grow taller. Normally, plants growing with an unshaded light source will grow straight up towards the sun because auxin is evenly distributed all around the shoot.
But when the light is heavily shaded and comes in from an angle, something interesting happens. Auxin starts to concentrate on the shaded side of the plant instead, and as a result, the cells on the sunny side stay the same size but the cells on the shaded side grow longer. This causes the plant to tip and grow towards the light.
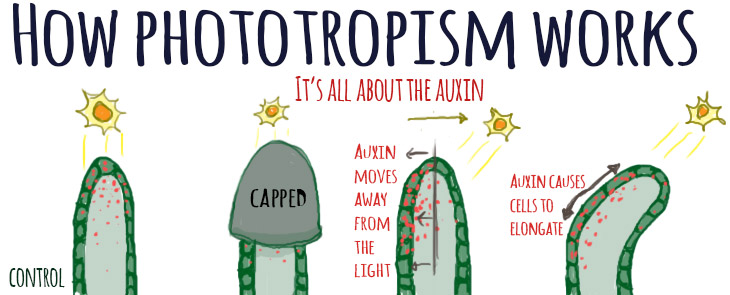
Auxin is primarily produced in the tips of the plants. This is why the plant grew straight up when you covered the tip with a cap—it couldn’t “see” the light anymore! The tips of the control seedlings and the seedlings with the bases covered could still sense the light, so they grew towards the sunlight.
Thanks to Charles Darwin and modern science, the mystery of how plants grow towards light was finally solved.
Learn more about phototropism:
To understand plant tropisms, you first have to understand plant hormones. We created an excellent page about Plant Growth Hormones here and here .
Related Topics
Choose one of the following categories to see related pages:.
- Experiments ,
Share this Page
Lindsay graduated with a master’s degree in wildlife biology and conservation from the University of Alaska Fairbanks. She also spent her time in Alaska racing sled dogs, and studying caribou and how well they are able to digest nutrients from their foods. Now, she enjoys sampling fine craft beers in Fort Collins, Colorado, knitting, and helping to inspire people to learn more about wildlife, nature, and science in general.
Plant Biology-boo
- Monocots vs Dicots Explained
- Phototropism Experiment
- Plant Diversity
- Plant Growth Hormones
- Plant Memory
- Transport in Plants
- Water Transport Experiment
- Why leaves change color
Science Newsletter:
Full list of our videos.
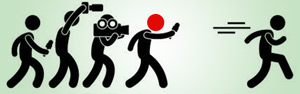
Teaching Biology?
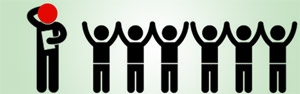
How to Make Science Films
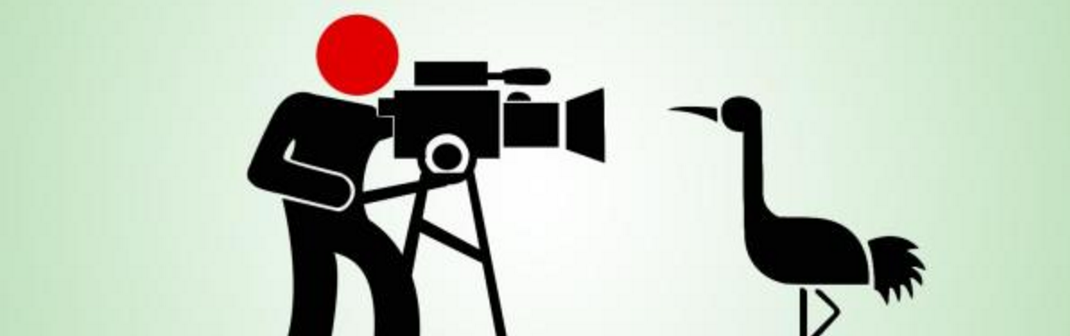
Read our Wildlife Guide
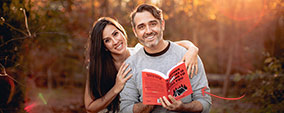
New From Untamed Science
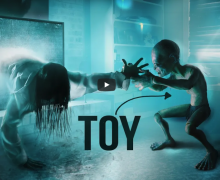
- Frontiers in Plant Science
- Plant Metabolism and Chemodiversity
- Research Topics
Light-Driven Redox Reactions Underlying Plant Metabolic Pathways in Changing Environments
Total Downloads
Total Views and Downloads
About this Research Topic
Signal transduction, redox-mediated enzyme regulation, and changes to the protein complexes involved in light energy harvesting and photochemical conversion are important aspects of light-driven metabolic adjustment. Furthermore, redox systems in plant cell compartments are differentially influenced by changes in light intensity and spectrum. Investigations into light-driven redox reactions occurring at the molecular level promise to influence rational redesigns of photosynthesis to sustainably meet current and future global demand for food and energy. This Research Topic will provide an updated overview of recent and innovative findings regarding different aspects of basic bioenergetics underlying metabolic pathways, with a particular emphasis on the mechanisms regulating photosynthesis from molecular, cellular, organismal, and ecological perspectives. Accordingly, we aim to collect original research manuscripts, methods papers, systematic reviews, mini-review perspectives, and opinions that focus on, but are not limited to, the themes listed below. Please note that all photosynthetic organisms fall within the scope of this collection. • New genome editing technologies for reengineering photosynthetic organisms; • Exploration and exploitation of natural genetic and epigenetic diversity of redox-active protein machinery associated with environmental adaptations; • Functional characterization of genes involved in photosynthetic light-harvesting, photochemical conversion, and regeneration of photosynthetic protein complexes; • Structural characterization of thylakoid-associated proteins from either the stromal side or the luminal side that are involved in the regulation and regeneration of protein complex dynamics under changing environmental conditions, be they biotic or abiotic; • Bioinformatics and modeling approaches to elucidating the regulatory networks underlying diurnal light cycles in photosynthetic organisms (plants and microbes); • Critical overviews of past, present, and future of photosynthetic research.
Keywords : redox reactions, plant metabolism, photosynthesis, bioenergetics
Important Note : All contributions to this Research Topic must be within the scope of the section and journal to which they are submitted, as defined in their mission statements. Frontiers reserves the right to guide an out-of-scope manuscript to a more suitable section or journal at any stage of peer review.
Topic Editors
Topic coordinators, submission deadlines, participating journals.
Manuscripts can be submitted to this Research Topic via the following journals:
total views
- Demographics
No records found
total views article views downloads topic views
Top countries
Top referring sites, about frontiers research topics.
With their unique mixes of varied contributions from Original Research to Review Articles, Research Topics unify the most influential researchers, the latest key findings and historical advances in a hot research area! Find out more on how to host your own Frontiers Research Topic or contribute to one as an author.

IMAGES
VIDEO
COMMENTS
Step by step instructions: Growing the plant(s) 1. Fill each container with soilless Water the container and set under medium to about 1 cm below 3. grow lights or in a sunny window. the top. Water daily or as needed. 2. Press 2 or 3 seeds about 2 cm deep Once the plants have sprouted and are into the soilless medium. 4.
A total of 83 seedlings (Light, n = 28; Light-Dark, n = 30; Dark, n = 25) were individually trained inside a Y-maze, where the fan [F] and the blue LED light [L] were always positioned together on ...
prove that plants will move toward the light. Ask students if they know what a maze is and if they have ever seen one, (Answers may include a corn maze, a hedge maze, or a puzzle maze drawn on paper.) Now tell students they are going to create a maze that a plant will have to solve in order to reach the light. Procedure
Materials needed for the plant: Small plastic plant container about 7 - 10 cm high (or recycled paper cups with a drainage hole in the bottom) Plant Light Shoebox Maze Experiment. Potting soil (soilless medium)Cotton balls or rockwool. 2 - 3 pole bean seeds per plant container (ensure that you use pole bean seeds which grow long and tall ...
Make your own plant light maze to see phototropism in action! Make sure to open up your box to check on your plant's movement through the maze (and to give it some water) every few days. Watch over time as your plant turns and bends to make it around the obstacles/s, towards the opening in the box and the light. Step Put your flower pot in the
Introduction. Photoinhibition often occurs when light energy is excessive, which reduces photochemical efficiency and even causes photooxidative system damage (Ma et al., 2015; Dias et al., 2018).Furthermore, low light intensity influences photosynthesis, which is central to plant productivity, and can therefore severely restrict plant growth (Zhu et al., 2014), and even death (Wang et al., 2021).
Step 2. Prepare your plant. We picked a bean because it grows pretty quickly. Plant the seed in a cup of dirt (about 1 inch under the surface) and add water so that the dirt is moist.You may want to plant several cups ahead of time to make sure the bean sprouts.Optional: Place the plant near a windowsill and wait a few days until a small plant ...
SP6: Constructing explanations. PLANT BEANS IN A JAR ( Week 1 ): Using the bowl of water, wet pieces of paper towels. Paper towels should be fully saturated but not dripping wet. Line a glass jar with these wet paper towels. Place approximately 3-4 bean seeds (e.g., lima or kidney beans) between the jar and moist paper towels.
1. Plant your seed - plant a bean seed in a cup of dirt (buried about 2.5cm deep. You may want to plant a few cups ahead of time to make sure the bean sprouts. 2. Water your plant. 3. Time to prepare your maze! Cut a hole in the top left corner of your box - this is the maze exit. 4.
Plant materials. The maize (Zea mays L.) inbred line Qi319, which is widely used in photosynthesis research, was grown in a greenhouse at 28 °C/23 °C (day/night) with a 16h/8h (light/dark) cycle, 60% humidity, and 400 μmol·m -2 ·s -1 light intensity.When the second leaves of the seedlings were fully expanded under normal light conditions (SNL), the aboveground portions of three ...
The effects of B light percentages on plant morphology have been previously reported in several studies [8,11,12,21,22,23,24,25]. In general, B light is sensed by the cryptochrome system, where under high irradiances or high levels of B light, plants exhibit shorter and stunted growth (For example [8,14,26]). It is also known that a total lack ...
Different colors of LED lights have different effects on the growth and development of diverse plants, and most of the research has mainly focused on red and blue light (Li et al., 2017).For example, blue light exposure has been reported to reduce leaf area and shoot dry weight of the Asteraceae plants, but to increase those of the Solanaceae plants (Sabzalian et al., 2014; Vu et al., 2014).
PLANT BEANS IN A JAR (Week 1): Using the bowl of water, wet pieces of paper towels. Paper towels should be fully saturated but not dripping wet. Line a glass jar with these wet paper towels. Place approximately 3-4 bean seeds (e.g., lima or kidney beans) between the jar and moist paper towels. Make sure each seed is visible.
(a) Light-emitting diode light(s) can sustain normal plant growth. Pioneer experiments on plant growth under red LEDs on lettuce were reported by Bula et al.[]Martineau et al. [] calculated that the amounts of dry matter per mole of artificial lighting gained by lettuce grown using red (650 nm) LEDs or high-pressure sodium lamps were identical, and Chang et al.
Light is the primary driving force for photosynthesis and thus dictates carbon assimilation, biomass production, and yield in plants. Light also plays an important role in a myriad of physiological and biochemical processes in plants, from eliciting specific gene responses to whole-plant phenomics. The lighting environment is especially important in controlled environment agriculture (CEA) as ...
Introduction. The shading stress or weak light condition is one of the common abiotic stresses in agricultural production, which restrains the growth of plants in some unfavorable cultivation practices, such as high planting density (Liu et al., 2010) and agroforestry compound systems (Zhang et al., 2018).The leaves are the main organs of plant photosynthesis that first detect changes in the ...
plants will move toward the light. Ask students if they know what a maze is and if they have ever seen one,. (Answers may include a corn maze, a hedge maze, or a puzzle maze drawn on paper.) Now tell students they are going to create a maze that a plant will have to solve in order to reach the light. PROCEDURE:
The Light Emitting Diode (LED) lights are extensively utilized in the cultivation of several plant species , especially horticultural plants due to their lower power consumption and higher ...
The photosynthetic capacity of leaves is determined by their content of nitrogen (N). Nitrogen involved in photosynthesis is divided between soluble proteins and thylakoid membrane proteins. In C4 plants, the photosynthetic apparatus is partitioned between two cell types: mesophyll cells and bundle sheath. The enzymes involved in the C4 carbon cycle and assimilation of nitrogen are localized ...
Darwin correctly concluded that plants are able to "see" light using the tips of the plant shoots, rather than through the stalks. It wasn't until a bit later that scientists figured out exactly why that was, though. It turns out that plants are able to grow by using hormones such as auxins and gibberellins. Auxin in particular tells ...
plants will move toward the light. Ask students if they know what a maze is and if they have ever seen one,. (Answers may include a corn maze, a hedge maze, or a puzzle maze drawn on paper.) Now tell students they are going to create a maze that a plant will have to solve in order to reach the light. PROCEDURE:
Maize Research. is working as a Scientist (Plant Breeding and. is a senior scientist (Plant pathology) of. September 1982 in Sarkuwa- 7 Baglung, Nepal. He is the. About the A uthors. is currently ...
Signal transduction, redox-mediated enzyme regulation, and changes to the protein complexes involved in light energy harvesting and photochemical conversion are important aspects of light-driven metabolic adjustment. Furthermore, redox systems in plant cell compartments are differentially influenced by changes in light intensity and spectrum. Investigations into light-driven redox reactions ...