Thank you for visiting nature.com. You are using a browser version with limited support for CSS. To obtain the best experience, we recommend you use a more up to date browser (or turn off compatibility mode in Internet Explorer). In the meantime, to ensure continued support, we are displaying the site without styles and JavaScript.
- View all journals
- My Account Login
- Explore content
- About the journal
- Publish with us
- Sign up for alerts
- Open access
- Published: 04 October 2021

AI-based design of a nuclear reactor core
- Vladimir Sobes 1 ,
- Briana Hiscox 2 ,
- Emilian Popov 2 ,
- Rick Archibald 2 ,
- Cory Hauck 2 ,
- Ben Betzler 2 &
- Kurt Terrani 3
Scientific Reports volume 11 , Article number: 19646 ( 2021 ) Cite this article
6541 Accesses
9 Citations
1 Altmetric
Metrics details
- Applied mathematics
- Computational science
- Energy science and technology
- Engineering
- Mathematics and computing
- Nuclear energy
- Power stations
- Scientific data
The authors developed an artificial intelligence (AI)-based algorithm for the design and optimization of a nuclear reactor core based on a flexible geometry and demonstrated a 3× improvement in the selected performance metric: temperature peaking factor. The rapid development of advanced, and specifically, additive manufacturing (3-D printing) and its introduction into advanced nuclear core design through the Transformational Challenge Reactor program have presented the opportunity to explore the arbitrary geometry design of nuclear-heated structures. The primary challenge is that the arbitrary geometry design space is vast and requires the computational evaluation of many candidate designs, and the multiphysics simulation of nuclear systems is very time-intensive. Therefore, the authors developed a machine learning-based multiphysics emulator and evaluated thousands of candidate geometries on Summit, Oak Ridge National Laboratory’s leadership class supercomputer. The results presented in this work demonstrate temperature distribution smoothing in a nuclear reactor core through the manipulation of the geometry, which is traditionally achieved in light water reactors through variable assembly loading in the axial direction and fuel shuffling during refueling in the radial direction. The conclusions discuss the future implications for nuclear systems design with arbitrary geometry and the potential for AI-based autonomous design algorithms.
Similar content being viewed by others
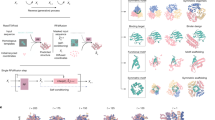
De novo design of protein structure and function with RFdiffusion
Joseph L. Watson, David Juergens, … David Baker
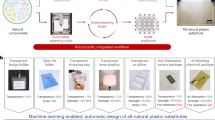
Machine intelligence-accelerated discovery of all-natural plastic substitutes
Tianle Chen, Zhenqian Pang, … Po-Yen Chen
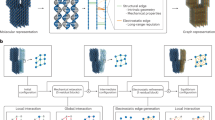
Prediction of DNA origami shape using graph neural network
Chien Truong-Quoc, Jae Young Lee, … Do-Nyun Kim
Introduction
The rapid development of advanced manufacturing and its application to advanced reactor design in the Transformational Challenge Reactor (TCR) program 1 have presented the opportunity to explore the potential revolutionary benefits of the arbitrary geometry design of nuclear systems. Nuclear engineering design is no longer bound to the simple geometries manufacturable by traditional methods, slabs, cylinders, and spheres (e.g., fuel plates, fuel pellets, fuel pebbles) 2 , 3 . However, the increased freedom of designing an arbitrary geometry system comes at the cost of an increased complexity in design optimization. Dimensionality quickly makes the design problem overwhelming for engineers. To address this issue, the authors implemented an artificial intelligence (AI)-based optimization algorithm and established a challenge problem to demonstrate the application.
The basis of the challenge problem is to determine the optimal geometric shape in the axial dimension of the cooling channels of a simplified reactor design’s full-core model. The reactor core used in this AI design optimization is based on a simplification of the design of the actual TCR core. The core is a right cylinder that is 1 m in diameter and 80 cm tall. Nine concentric rings of hexagonal assemblies surround a central hexagonal assembly. The fuel compact of traditional TRISO particles is an annular design with a 2.5 cm outer diameter, and sits in the middle of each 5 cm wide (flat to flat) hexagonal assembly. There are cooling channels inside the annular fuel, and an yttrium-hydride the moderator surrounds the outside of the fuel annulus. The center assembly in the core is pure moderator, uncooled and unfueled. The helium coolant flows from the bottom to the top in all the fueled assemblies. Figure 1 presents a core schematic.
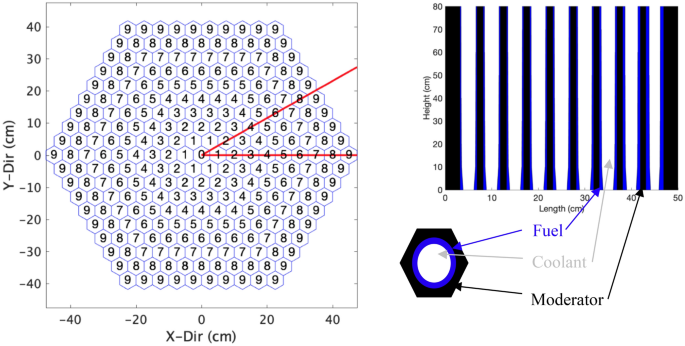
The top view of the core is shown on the left with the nine radial assembly rings labeled. Only the symmetric 1/12 segment inside the red lines is modeled. A horizontal slice of one assembly and an axial cross section of the optimized core configuration is shown on the right. Created using MATLAB R2020b, www.mathworks.com .
The design space encompassed the axial profile of the coolant channel in each of the nine assembly radial rings. That is, all the assemblies in each ring had the same axial coolant channel profile, but the coolant channel profile was different for each radial assembly ring. The geometry optimization was parametrized by a set of coolant channel radii. Each of the nine assembly radial rings had an independent set of nine coolant channel radii which spanned the 80 cm height of the core in 10 cm vertical segments. The radium of the coolant channel was a piece-wise linear function connecting these nine radii for each assembly. The maximum coolant channel radius was constrained to be greater than 1 mm and 2.4 cm (1 mm less than the fuel outer radius).
The objective for this core design was to minimize the temperature peaking factor across each 10 cm vertical segment (eight making the full axial height) of each assembly while maintaining a critical core configuration. The engineering justification for this objective function was to minimize the mechanical stresses due to temperature gradients in the components, although no thermomechanical analyses were performed in this initial study. Specifically, the objective function is defined as:
where \(T\left(x,y,z,\Phi \right)\) is the temperature at the location \(\left(x,y,z\right)\) and design parameters \(\Phi\) .
The challenge problem inherently requires multiphysics modeling between neutron transport and thermofluidics. The problem is further convoluted because the cooling channel radius (axially variable) simultaneously controls everything about the heat transfer process and the amount of fuel at each axial core level because the fuel annulus outer diameter is fixed. The predictive simulation of candidate configurations requires computationally intensive modeling. The authors used a Monte Carlo-based code for neutron transport coupled to a computational fluid dynamics (CFD) code for the thermofluidics. With a large potential design space to explore, it was impractical to evaluate all of the candidate designs with the full fidelity physics. Therefore, the authors developed a machine learning (ML)-based multiphysics emulator that was designed to run on Summit, Oak Ridge National Laboratory’s (ORNL’s) GPU-based high-performance computing (HPC) system 4 . By training the ML-based emulator, the authors achieved errors as low as a few percent, which allowed them to quickly and reliably sample thousands of candidate designs on Summit. Only the most promising candidate designs were tested with the full-fidelity physics simulations. The emulator was updated, and convergence on the optimal design was achieved in only a few iterations.
The “ Background ” section reviews of some of the previous attempts at AI-based nuclear reactor design and optimization that did not leverage the advantages of arbitrary geometry. The section also discusses designing ML-based emulators for computationally intensive full-fidelity modeling software. The “ Methods ” section describes the overall workflow of the authors’ approach and presents the details of the full-fidelity modeling, the design of the emulator, and the optimization approach used on Summit. The “ Results ” section presents and discusses the optimal design solution to the challenge problem. Lastly, the “ Discussion ” section concludes with the authors’ outlook on the future of nuclear systems design in arbitrary geometry and discusses how the AI-based nuclear systems design approach can supplement the toolbox of nuclear industry designers.
Today, reactor cores are built from industrial materials that represent regular (i.e., usually cylindrical or plate) component geometries. The reactor cores have a periodic structure; for example, one component (i.e., core fuel element) is repeated multiple times to create the entire core. This approach results in geometrically similar fuel elements with a regular shape 5 . The fuel volumetric (per unit volume of core) content is spatially uniform. This limitation causes complications and results in fuel usage with different enrichment to efficiently design the entire core, minimizing power and thermal gradients. Similar observations can be made regarding the hydraulic design. The core is cooled to remove heat and generate power. The periodic repeatable fuel structure imminently produces the same regular coolant channel configuration (e.g., all coolant channels are the same and will produce the same level of cooling under the same conditions). The background of this research assumes an additively manufactured core in which the fuel and cooling channel geometries have practically unlimited spatial degrees of freedom. This is the primary difference explored in this work, and it might bring unforeseen performance that could be hidden from designers but easily discoverable for an unbiased AI algorithm.
Previous attempts at AI-based design and optimization of nuclear reactor cores are found in other works; e.g. References 6 , 7 , 8 , 9 , 10 , 11 , 12 , 13 . One common theme among these earlier works is that the optimization problem is posed as a combinatorial problem with fixed geometry rather than as an optimization over continuously variable geometry parametrization. One example is the fuel shuffling during the reloading of a boiling water reactor core. Genetic algorithms have been the predominant choice of AI algorithms for the combinatorial optimization problem. When more continuous variable geometries were studied, more structured optimization approaches were used 14 . A recent work considered the nuclear systems design by using genetic algorithms created from scratch rather than an optimization of an existing configuration 15 . However, arbitrary geometry could only be considered through a voxel representation. Lastly, the authors had published on the framework for an arbitrary geometry optimization of nuclear systems and gave some demonstrations on simplified challenge problems 2 . This work is the application of that framework to a nuclear reactor full core.
The mathematical formulations and their solutions for the underlying multiphysics phenomena that occur in the reactor core are well-known. For this work, the focus in on solutions of the Boltzmann transport equation, which will be the driving term to Poisson’s equation coupled with the Navier–Stokes equations. The solution to this set of coupled equations in nuclear engineering is tied up in several complex computer codes and is computationally intensive. Therefore, an efficient multiparameter optimization search using high-fidelity physics models is prohibitively expensive. In this work, the authors explored the automatic construction of physics-informed ML methods by using emulators with validation from very sparse sampling of the predictive high-fidelity physics simulations. The physics-informed emulators are based on a steady-state reduced-order model through Gaussian kernel convolution that allows for a fast evaluation on a single GPU 16 , which is suitable for ideal scalability in the search over the vast design space.
Surrogate modeling is a well-used method in science and engineering 17 . The fundamental approach is to approximate quantities of interest from complex systems by using cost-effective and accurate surrogates that otherwise could only be measured or simulated at very high cost, if at all. Within the context of design optimization, building cost-effective and accurate surrogates enables the exploration of complicated design space, accelerating the process of finding the best designs for given loss functions. The surrogate modeling method that the authors developed in this work falls under the category of multi-fidelity surrogate modeling. The authors merged the information generated by the dense sampling of reduced-order modeling with sparse full physics simulation via Gaussian processes (GPs) 18 . This work uniquely developed reduced-order models of the neutronics and thermofluidic physics that were designed for a fast evaluation on a single GPU. Thus, it is possible to evaluate millions of designs by using GPU-based HPC systems, such as Summit 4 .
Optimization work flow: inner and outer loops
The authors identified two categories of tasks necessary for successfully developing a holistic AI-based approach to computationally optimizing nuclear systems. The tasks are separated into the inner computational loop and the outer optimization loop. The inner computational loop involves the full-fidelity physics simulation of the candidate designs needed to create the training data for the multiphysics ML-based emulator. The outer optimization loop begins with training the emulator based on the results generated with the full-fidelity physics calculations, and design parameters are chosen from a random sample of the design space. The outer optimization loop is the AI model for the design space that is updated based on massively parallel evaluations of the multiphysics emulator on thousands of candidate geometries. Figure 2 illustrates the workflow. The outer loop can be thought of as an adaptive sampling method, ubiquitous in computation design 17 , where each iteration focuses on a smaller design space that minimizes the given loss function.
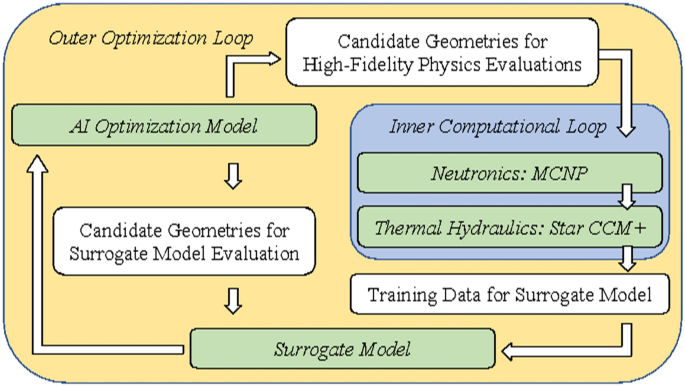
Illustration of the AI optimization workflow. Created using Microsoft PowerPoint, version 16.52, www.microsoft.com .
Inner computational loop
The neutronic modeling for this project was conducted in the Monte Carlo N-Particle code 19 . This code was chosen due to its ability to calculate power in nonfuel materials. This allowed for a very accurate power density profile in all materials. To save on computational time, the model was 30° of the full core (1/12) with reflective boundary conditions (i.e., instead of the full 360°). Because of the intrinsic symmetry and core optimization variables, this model represents a full-core model. The model was run with four tally meshes to account for the energy deposition in a cell from neutrons and photons in the fuel and moderator materials. A tally was produced for each mesh bin. The mesh bin density was 504 in the x direction, 252 in the y direction, and 20 in the z direction. Therefore, the lengths of each mesh bin were 0.1, 0.1, and 4 cm. These sizes were determined because they have a reasonable computational cost neutronically and thermofluidically, and meshing studies determined that they were sufficiently fine to achieve good resolution on the fuel annulus and coolant channels.
There were 750 cycles run with 10,000 particles in each cycle. The first 50 cycles were inactive which means they were not taken into account for the determination of k-effective, flux, or reaction rates. It is necessary to have inactive cycles in the beginning of a Monte Carlo simulation to converge the fission source. The average standard deviation of k-effective was 0.00024 and the maximum was 0.00030. The power density was normalized to maintain a constant core power of 3 MW. MCNP was run in “mode N P” which accounts for neutron-induced photons. This is necessary for the photon-heating tallies.
The thermofluidics model developed for this work relies on numerical methods (e.g., temporal and spatial discretization) and physical models (e.g., turbulent flow, conjugate heat transfer) to predict the temperature and flow distribution in the geometry of interest. The inherent assumptions of these methods and models must be quantified to ensure the correctness and accuracy of the results. In view of the diverse geometry configurations of the optimized designs, an accurate prediction of temperature distribution in the component is necessary. The thermofluidic model must be capable of computing a conjugate (i.e., solid fluid) heat transfer in arbitrary geometric shapes. To achieve this, a CFD approach was taken, and the commercial software STAR-CCM+ was used 20 . This method allows complex surfaces to be discretized with finite volume techniques, as well as allows the interface between the solid structure and the coolant—gas, in this case—to be properly defined. On the solid side, a thermal diffusion of heat with a volumetric heat source is computed to determine the temperature distribution. The heat generated by nuclear fission is deposited in the core element according to the volumetric power distribution supplied by the reactor physics calculation.
On the fluid side, a Reynolds averaging of the velocity vector field was employed within the finite volume formulation. Since the assumed flow is highly turbulent, a two-equation model of turbulence, realizable k-epsilon, was used. This model is better than the standard k-epsilon model for many applications, including rotational and shear flows, and it generally gives answers that are at least as accurate 21 . The near-wall velocity field is resolved with the two-layer all wye (Y) plus method 20 . All these models accurately predict the wall heat transfer, which is critical for the proper resolution of component temperature field.
To model the geometry variation necessary for running the suite of optimization codes, a geometry parameterization method was used. Within the CFD computation, the geometry is regenerated automatically every time a new combination of parameters is tried. The software allows the computational domain to be modified—both in its geometry and discretization—without user intervention. This is achieved by automating the computing process with Java drivers. The approach is fully integrable in an autonomous workflow within the entire optimization suite.
For the thermofluidic calculation, the domain was discretized with a variable resolution but with at least four elements in the radial direction across fuel. It was meshed with a polyhedral mesh with a base dimension determined by the smallest fuel element. Because the geometry varies by fuel and channel sizes, the number of elements per each case is different and usually stays below 7 million. Some specific cases with thin fuel might increase the element count to 20 million, but this is rare. The calculations were run in parallel on 16 processes, and most run time is spent on grid generation. The average clock time for a single run is around 2 h. The cases are run until the convergence of momentum and energy residuals for at least three orders of magnitude is achieved. Sensitivity on mesh and residuals convergence was performed to allow for temperature accuracy of less than 1° to be achieved. The employed modeling approach relies on component physics validation which is part of the software qualification process implemented at ORNL. The flow and heat transfer solutions, and the corresponding modeling techniques, were initially tested and verified by standard test problems. The models will be further tested against integral experiments after such are carried out as part of a comprehensive testing program.
Figure 3 illustrates a typical result from the thermofluidic solution. Contours of temperatures are shown at three axial levels in the core: low (15 cm), middle (45 cm), and top (75 cm). The highest temperature is reached on the fuel-moderator boundary because the design does not involve extra moderator cooling and because the power deposited in the moderator is removed only through fuel-cooling channels. The radial variation of cooling channels is clearly visible, as defined by the optimization algorithm. Channels are larger in the central part and smaller at the periphery where the power density is lower and less coolant is needed. The average pressure drop and fuel temperature and the maximum fuel temperature difference were also calculated and are provided for reference. The input power density is plotted on the left side of Fig. 3 at the same axial positions. The simulation uses power deposition in the fuel and moderator. The power plot gives some impression for the fuel thickness variation (light blue color) with more fuel toward the periphery and less in the middle of core. Although not evident from the limited axial locations plotted, the fuel and channel sizes also vary axially as eight piece-wise linear sections (Fig. 1 ).
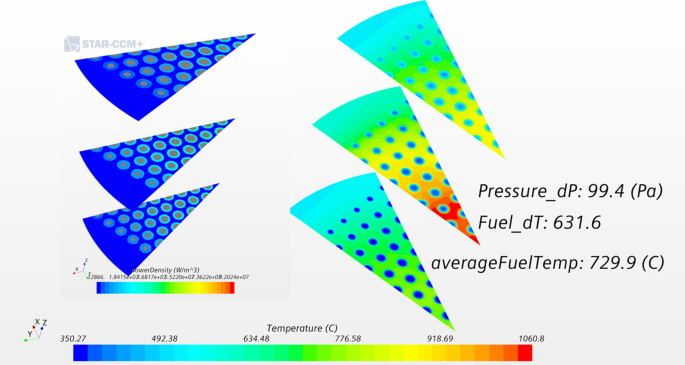
Temperature field from the thermofluidic solution. Three axial sections are plotted at lower, middle, and upper core element levels. They show the temperatures in the coolant, fuel, and moderator. On the left, the power input to the fuel and moderator is provided for reference. Both fuel and channel sizes vary in the radial direction. Created using Star-CCM+, version 2020.1, www.plm.automation.siemens.com/global/en/products/simcenter/STAR-CCM.html .
Outer optimization loop
Simulation-based computational design can quickly become an intractable problem, depending on the size of the computational design space and computational complexity of the design simulation. The authors developed reduced-order surrogate models for neutronics and thermofluidics that can quickly sample hundreds of thousands of geometries on Summit. By using the combination of surrogate modeling and sparse validation with correction from full-physics simulation, the authors were able to train GP ML methods to accurately predict optimal designs. On average, one reactor core design takes ~ 150 s for the reduced-order surrogate model to simulate on a single Summit GPU. This time includes all setup costs and data movement. With six GPUs per node, the authors were able to test ~ 150 reactor geometries per hour per node. Generally, the surrogate is around 95% accurate compared with full physics simulations that use relative least square error measure on the objective function for this problem. Also, the surrogate model generally struggles the most at the inlet region. For the core challenge problem, Summit simulated ~ 10,000 different geometries in combination with ~ 100 full physics simulations in four iterations to determine an optimal design. We provide the code in the supplemental materials for this paper.
The outer loop AI model is based upon Gaussian processes, which are a kernel-based machine learning method that provides an efficient method for ML applicable to physics-oriented problems in engineering sciences. Specifically, given a set,
of training data the loss can be determined for any parameter set. Here, x is the position vector, and \({p}_{i}\) is the parameter vector for the i th design. The functions are the power \({P}_{i}\) , component temperature \({T}_{i}\) , fractional coolant indicator \({V}_{i,c}\) , fractional fuel indicator \({V}_{i,f}\) , and fractional moderator indicator \({V}_{i,m}\) for the N simulated training sets. The loss \({L}_{i}\) for any design is defined to be the standard deviation of temperature for every domain with positive fuel indicator. The last item in this collection is the error estimation of the loss \({\sigma }_{i}\) . The loss of any design \(p\) is predicted by using the kernel-based ML method defined as:
where kernel function, \(k\left(p,{p}_{i}\right)={e}^{-\frac{1}{2}{\| p-{p}_{i}\| }^{2}}\) is used. The coefficients of the kernel-based ML are found by solving
for \(j=1,...,N\) training sets, where L j losses are known. The matrix elements are given as \({K}_{i,j}=k\left({p}_{i},{p}_{j}\right)\) for \(1\le i,j\le N\) and the coefficient vector \(c=\left({c}_{1},...,{c}_{N}\right)\) .
The data from the full-fidelity physic model are augmented with a set of M emulated models:
The ML method assumes that the full fidelity physical models are exact, or \({\sigma }_{i}=0\) for \(i=1,...,N\) . In the case of \(i=N+1,...,N+M\) , a low-resolution approximation of the physics is used to estimate the temperature function \(\tilde{T }\) by solving:
where \({\upsilon }_{i}\left(x\right)\) is a flow field, \(\frac{\partial {\tilde{T }}_{i}\left(x\right)}{\partial x}\) is the temperature gradient along the flow direction, and \({\alpha }_{c}\) , \({\alpha }_{f}\) , and \({\alpha }_{m}\) are constants. For any design \(p\) , the flow field is calculated based on the volumetric rate of coolant. The flow field is zero in the solid material of the reactor. The flow field and constants \({\alpha }_{c}\) , \({\alpha }_{f}\) , and \({\alpha }_{m}\) are calculated such that \({\sum }_{i=1}^{N}{\| {T}_{i}\left(x\right)-{\tilde{T }}_{i}\left(x\right)\| }_{2}\) is minimized, where T i (x) is the training set temperatures.
When the data are augmented, the Gaussian process is calculated by:
where the kernel function, \(k\left(p,{p}_{i}\right)={e}^{-\frac{1}{2}{\| p-{p}_{i}\| }^{2}}\) is used. The coefficients of the kernel-based ML are found by solving
for \(j=1,...,N+M\) and \({\sigma }_{i}=0\) for \(i\le N\) , where the matrix elements are given as \({K}_{i,j}=k\left({p}_{i},{p}_{j}\right)\) for \(1\le i,j\le N+M\) , the coefficient vector \(c=\left({c}_{1},...,{c}_{N+M}\right)\) , and σ i is an estimate for the error in the emulation.
The quantitative objective function for the challenge problem’s optimization design was to minimize the temperature peaking in each 10 cm axial section (eight total for the full-core height) of each assembly in the core. From single-assembly simulations, the average numerical value of the objective function for a conventional design with axially uniform coolant channels of one radius was 842.1 ℃. The optimal design results in a final value of the objective function of 291.35 ℃; a 3× improvement in the objective function is achieved through the AI-based optimization of the geometry of the cooling channels when compared to the constant-cooling-channel-radius design. Figure 4 presents the visualization of the calculated temperature distribution in the core before and after the optimization.
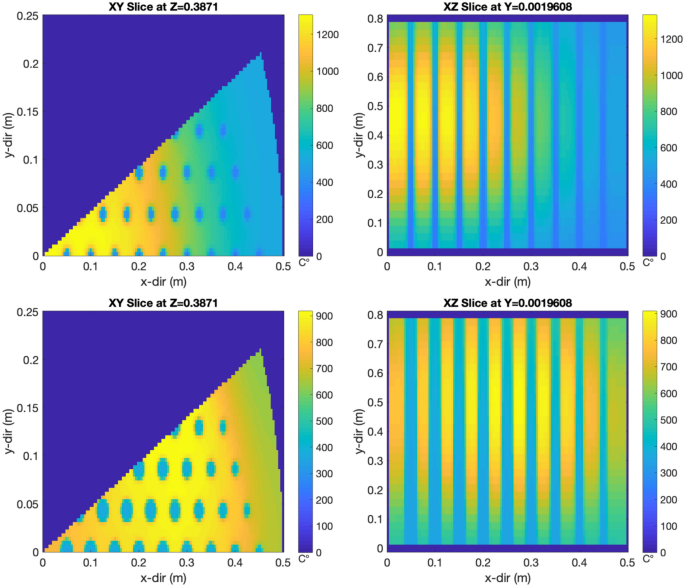
Visual representation of the temperature distribution in the original (top) and optimized (bottom) cores. The left two figures present a radial slice at an axial location of 38.7 cm out of a total core height of 80 cm. The right two figures present an axial slice through the core. Created using MATLAB R2020b, www.mathworks.com .
Figure 5 presents a physical interpretation of the optimization results by plotting the volume of the fuel in each assembly and the heat-exchange area for the cooling channels. The initial configuration with axially uniform cooling channels in all assemblies is given in black. This presents the optimal solution for a uniform cooling channel configuration. An intermediate stage of the optimization process is shown in blue, and the final, optimal design is presented in green. The intermediate result can be identified by the non-smooth behavior of the volume and surface plots across the nine radial assembly rings, whereas the converged solution displays the physically expected smooth behavior. Furthermore, there is a trade-off between the increased heat-exchange area of the cooling channel and the reduced fuel volume. Both contribute in the same direction to reducing the temperature peaking in the middle of the core that is observed with the uniform cooling channel design.
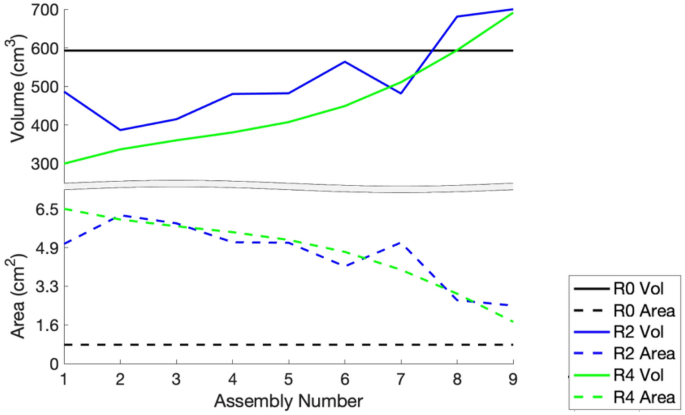
Plot of the fuel volume per assembly (top) and the heat-exchange area for the cooling channel in each assembly (bottom). The three different colors represent different iterations of the optimization algorithm: initial (R0) and two later iterations (R2 and R4). Here, R0, R2, and R4 are shorthand for iteration round zero, two, and four, respectively. Created using MATLAB R2020b, www.mathworks.com .
Although the optimal configuration can be justified from an engineering analysis perspective, the result is far from trivial. The right side of Fig. 1 , which plots the axial cross section of the optimal design, shows that the profile of the cooling channels changes in two dimensions: axially with the height of the core and radially across the different assembly rings. The shape of the cooling channels is also unique for each of the radial assembly rings rather than being a scaled or translated version of each other. The top plot in Fig. 5 also shows a significant reduction in the fuel volume needed to maintain the reactor critical at a fixed power level compared with the traditional, uniform design. Lastly, the extension of this result is that the reactor can be operated at higher power levels for the same amount of fuel and peak fuel temperature limits.
Summary of accomplishments
This paper presents the results for the AI-based design optimization of a full nuclear reactor core with arbitrary geometry cooling channels. To accelerate the optimization space search, the authors developed a ML-based multiphysics emulator capable of running efficiently on Summit. The authors demonstrated how an AI-based optimization algorithm can efficiently sample the vast and continuous search space of arbitrary geometry to find the optimal solution with significant performance improvement. In the established challenge problem, the authors demonstrated a 3× improvement in the performance metric of reducing temperature peaking factors across the core in the radial and axial directions.
Future for nuclear design with arbitrary geometry
The authors envision a rapidly developing and promising future for nuclear systems design with arbitrary geometry. Arbitrary geometry enabled through advanced manufacturing provides a vast variety of unexplored opportunities in nuclear systems design. Historically, many engineering objectives in nuclear reactor design were met through creative combinatorial solutions of fuel loading axially and radially. Arbitrary geometry allows researchers to explore alternative solutions to these engineering challenges. Furthermore, combining variable fuel loading with elements of arbitrary geometry to maximize nuclear reactor safety and economics is an exciting opportunity.
Future for ML-based surrogate models for rapid design evolution
The second exciting opportunity that could result from this work is the rapid design evolution that can be achieved with ML-based surrogate models, as presented in this work. The authors demonstrated that it is possible to construct ML-based surrogate models capable of capturing a large percentage of the system physics but that can be evaluated to predict the performance of candidate designs at a fraction of the computational time. This step is absolutely necessary to progress to searching larger and larger design spaces that allow for more complex nuclear system geometries.
Future for AI-based nuclear design
The authors do not anticipate that the AI-based nuclear systems design will completely replace human designers but rather anticipate that AI-based design will become one of the main tools of the human designer. In this case, the way in which engineers think about the design problem must shift. The new focus must be to carefully craft the parameters of the optimization problem and establish the objective and constraints. The accurate formulation of the objectives will be vital, especially finding the right balance between multiple objectives. The parameters of the optimization must be chosen very carefully to reduce the possible design space as much as possible while maintaining enough flexibility to allow for significant performance improvements.
Although many challenges undoubtedly still remain, the combination of (1) arbitrary geometry enabled by advanced manufacturing, (2) ML-based surrogate models for fast and predictive computational evaluation, and (3) AI-based optimization algorithms form a very exciting future for nuclear design with the potential to discover revolutionary changes in the safety, efficiency, and economy of nuclear systems helping contribute to safer and cleaner energy for the world.
Oak Ridge National Laboratory. Transformational challenge reactor program. https://tcr.ornl.gov/ (2021). Accessed 17 August 2021.
Sobes, V., et al . Artificial intelligence design of nuclear systems empowered by advanced manufacturing. In PHYSOR 2020—Transition to a Scalable Nuclear Future , Cambridge, United Kingdom, March 29–April 2, 2020.
B. R. Betzler, B. J. Ade, et al . Advanced manufacturing for nuclear core design. In PHYSOR 2020—Transition to a Scalable Nuclear Future , Cambridge, United Kingdom, March 29–April 2, 2020.
Oak Ridge Leadership Computing Facility, “Summit: America’s Newest and Smartest Supercomputer,”. https://www.olcf.ornl.gov/Summit/ (2021). Accessed 17 August 2021.
Betzler, B. R. et al. Advanced Manufacturing for Nuclear Core Design (Oak Ridge National Laboratory, 2019).
Book Google Scholar
Wilding, P. R., Murray, N. R. & Memmott, M. J. The use of multi-objective optimization to improve the design process of nuclear power plant systems. Ann. Nucl. Energy 137 , 107079. https://doi.org/10.1016/j.anucene.2019.107079 (2020).
Article CAS Google Scholar
Pereira, C. M. N. A. & Lapa, C. M. F. Coarse-grained parallel genetic algorithm applied to a nuclear reactor core design optimization problem. Ann. Nucl. Energy 30 (5), 555–565. https://doi.org/10.1016/S0306-4549(02)00106-8 (2003).
Pereira, C. M. N. A., Schirru, R. & Martinez, A. S. Basic investigations related to genetic algorithms in core designs. Ann. Nucl. Energy 26 (3), 173–193. https://doi.org/10.1016/S0306-4549(98)00036-X (1999).
Jayalal, M. L., Ramachandran, S., Rathakrishnan, S., Satya Murty, S. A. V. & Sai Baba, M. Application of genetic algorithm methodologies in fuel bundle burnup optimization of pressurized heavy water reactor. Nucl. Eng. Design 281 , 58–71. https://doi.org/10.1016/S0306-4549(98)00036-X (2015).
Pazirandeh, A. & Tayefi, S. Optimizing the fuel management in a VVER-1000 reactor using an artificial neural network. Ann. Nucl. Energy 42 , 112–118. https://doi.org/10.1016/S0306-4549(98)00036-X (2012).
Zameer, A., Mirza, S. M. & Mirza, N. M. Core loading pattern optimization of a typical two-loop 300MWe PWR using simulated annealing (SA), novel crossover genetic algorithms (GA) and hybrid GA(SA) schemes. Ann. Nucl. Energy 65 , 122–131. https://doi.org/10.1016/j.anucene.2013.10.024 (2014).
Gomez-Fernandez, M. et al. Status of research and development of learning-based approaches in nuclear science and engineering: A review. Nucl. Eng. Des. 359 , 110479. https://doi.org/10.1016/j.nucengdes.2019.110479 (2020).
Liu, Z., Wang, J., Tan, S., Qiao, S. & Ding, H. Multi-objective optimal design of the nuclear reactor pressurizer. Int. J. Adv. Nucl. Reactor Design Technol. 1 , 1–9. https://doi.org/10.1016/j.jandt.2019.09.001 (2019).
Article Google Scholar
Betzler, B. R., Chandler, D., Cook, D. H., Davidson, E. E. & Ilas, G. Design optimization methods for high-performance research reactor core design. Nucl. Eng. Des. 352 , 110167. https://doi.org/10.1016/j.nucengdes.2019.110167 (2019).
J. Pevey, O. Chvala, S. Davis, V. Sobes, W.Hines Genetic algorithm design of a coupled fast and thermal subcritical assembly. Nucl. Technol. 206 (4) (2020).
A. Archibald, V. Sobes, B. Hiscox, E. Popov, et al . Physics based machine learning for HPC computational design. In Conference on Data Analysis 2020 , Santa Fe, New Mexico, February 26, 2020.
Forrester, A., Sobester, A. & Keane, A. Engineering Design Via Surrogate Modelling: A Practical Guide (Wiley, 2008).
Williams, C. K. I. & Rasmussen, C. E. Gaussian Processes for Machine Learning Vol. 2 (MIT Press, 2006).
MATH Google Scholar
MCNP—A General Monte Carlo N-Particle Transport Code, Version 5 , Los Alamos National Laboratory, Vol. I, 2–71 ( 2–80 ) (2005).
Siemens. Simcenter STAR-CCM+ software. https://www.plm.automation.siemens.com/global/en/products/simcenter/STAR-CCM.html .
ANSYS, Inc. ANSYS-12.0 Theory Guide (2009).
Download references
Acknowledgements
This manuscript has been authored by UT-Battelle, LLC, under contract DE-AC05-00OR22725 with the US Department of Energy (DOE). The US government retains and the publisher, by accepting the article for publication, acknowledges that the US government retains a nonexclusive, paid-up, irrevocable, worldwide license to publish or reproduce the published form of this manuscript, or allow others to do so, for US government purposes. DOE will provide public access to these results of federally sponsored research in accordance with the DOE Public Access Plan ( http://energy.gov/downloads/doe-public-access-plan ). This work was funded by the U.S. Department of Energy Office of Nuclear Energy Transformational Challenge Reactor program. This research used resources of the Oak Ridge Leadership Computing Facility at the Oak Ridge National Laboratory, which is supported by the Office of Science of the U.S. Department of Energy under Contract No. DE-AC05-00OR22725.
Author information
Authors and affiliations.
University of Tennessee, Knoxville, USA
Vladimir Sobes
Oak Ridge National Laboratory, Oak Ridge, USA
Briana Hiscox, Emilian Popov, Rick Archibald, Cory Hauck & Ben Betzler
Ultra Safe Nuclear Corporation, Oak Ridge, USA
Kurt Terrani
You can also search for this author in PubMed Google Scholar
Contributions
V.S.: conceptualization; formal analysis; investigation; methodology; project administration; supervision; visualization; writing—original draft; writing—review and editing. B.H., E.P.: conceptualization; data curation; formal analysis; investigation; methodology; software; validation; visualization; writing—original draft; writing—review and editing. R.A.: conceptualization; data curation; formal analysis; investigation; methodology; resources; software; validation; visualization; writing—original draft; writing—review and editing. C.H.: conceptualization; formal analysis; investigation; methodology; visualization. B.B., K.T.: conceptualization; funding acquisition; project administration; resources; supervision; writing—review and editing.
Corresponding author
Correspondence to Vladimir Sobes .
Ethics declarations
Competing interests.
The authors declare no competing interests.
Additional information
Publisher's note.
Springer Nature remains neutral with regard to jurisdictional claims in published maps and institutional affiliations.
Supplementary Information
Supplementary information., rights and permissions.
Open Access This article is licensed under a Creative Commons Attribution 4.0 International License, which permits use, sharing, adaptation, distribution and reproduction in any medium or format, as long as you give appropriate credit to the original author(s) and the source, provide a link to the Creative Commons licence, and indicate if changes were made. The images or other third party material in this article are included in the article's Creative Commons licence, unless indicated otherwise in a credit line to the material. If material is not included in the article's Creative Commons licence and your intended use is not permitted by statutory regulation or exceeds the permitted use, you will need to obtain permission directly from the copyright holder. To view a copy of this licence, visit http://creativecommons.org/licenses/by/4.0/ .
Reprints and permissions
About this article
Cite this article.
Sobes, V., Hiscox, B., Popov, E. et al. AI-based design of a nuclear reactor core. Sci Rep 11 , 19646 (2021). https://doi.org/10.1038/s41598-021-98037-1
Download citation
Received : 07 April 2021
Accepted : 02 September 2021
Published : 04 October 2021
DOI : https://doi.org/10.1038/s41598-021-98037-1
Share this article
Anyone you share the following link with will be able to read this content:
Sorry, a shareable link is not currently available for this article.
Provided by the Springer Nature SharedIt content-sharing initiative
By submitting a comment you agree to abide by our Terms and Community Guidelines . If you find something abusive or that does not comply with our terms or guidelines please flag it as inappropriate.
Quick links
- Explore articles by subject
- Guide to authors
- Editorial policies
Sign up for the Nature Briefing newsletter — what matters in science, free to your inbox daily.

- Reference Manager
- Simple TEXT file
People also looked at
Editorial article, editorial: experimental and simulation research on nuclear reactor thermal-hydraulics.
- 1 Department of Nuclear Engineering and Technology, School of Energy and Power Engineering, Chongqing University, Chongqing, China
- 2 Department of Engineering Physics, University of Wisconsin-Madison, Madison, WI, United States
- 3 Helmholtz-Zentrum Dresden-Rossendorf, Dresden, Germany
Editorial on the Research Topic Experimental and simulation research on nuclear reactor thermal-hydraulics
The researches on nuclear reactor thermal-hydraulics have achieved outstanding progresses in the past decades. In recent years, basic research on multiphase flow dynamics and corresponding measurement technology, as well as preliminary research on Gen IV reactors based on experiments and simulations are attracting more and more attention. However, the inside complicated physics and outside extreme conditions will also bring risks and challenges to the development of nuclear industry.
Prof. Liangming Pan from Chongqing University held the first annual academic meeting of the branch of Nuclear Reactor Thermal-Hydraulics and Fluid Mechanics of Chinese Nuclear Society in October 2021. Several high-quality papers were selected from this conference. Therefore, Prof. Pan proposed the Research Topic “ Experimental and Simulation Research on Nuclear Reactor Thermal-Hydraulics ” in the journal Frontiers in Energy Research . This research topic aims to promote the novel experimental and numerical investigations on relevant issues refer to nuclear reactor thermal-hydraulics, which is of great significance to the system optimization and safety evaluation of nuclear power plants. Finally, 14 articles were collected and published in this topic, covering the experimental and numerical research of thermal hydraulic problems in light water reactors to supercritical coolant reactors.
Most papers are relevant to the thermal hydraulic problems in light water reactors. Li et al. have contributed an article: “ Research on Countercurrent Flow Limitation in Reactor Hot Leg at the Loss of Coolant Accident-Thermal hydraulic Calculation with System Code RELAP5 ”. Meng et al. have presented an article entitled: “ Research of Thermal Hydraulic Conditions Effect on PWR CIPS Risk ”. Yu et al. have presented an article: “ An Innovative Investigation on Fluid-to-Fluid Modeling of Post-Dryout Heat Transfer in Thermal Energy Systems ”. Zhu et al. have contributed an article: “ Comparison of Intergroup Mass Transfer Coefficient Correlations in Two-Group IATE for Subcooled Boiling Flow ”. Ding et al. have contributed their research relevant to: “ Experimental Research of Flow Distribution at Reactor Core Inlet of ACP100 ”. Li et al. have presented article in title of “ Experimental Study on Stratification Morphology of the Molten Pool during Severe Accident ”.
Several research papers are about the supercritical coolant. Zhang et al. have contributed an article: “ Experimental Study on Prototype of Printed Circuit Heat Exchanger ”. Zhu et al. have presented an article entitled: “ Dynamic Characteristic Study of Supercritical CO 2 Recompression Brayton Cycle System ”. Min et al. have presented their research article of: “ Study of Supercritical CO 2 Physical Property Calculation Models ”.
Several articles are related to the high temperature gas-cooled reactor. Qin et al. have presented an article entitled: “ Numerical Investigation of Hot Helium Flow Homogenizer on Inter-Unit Flow Rate Uniformity of HTGR Once through Steam Generator ”. Qu et al. have contributed an article: “ Characteristics Analysis of Combined Cycle Coupled With High Temperature Gas-Cooled Reactor Based on Progressive Optimization ”.
Meanwhile, there are several articles about other issues. Wang et al. have contributed the research paper of: “ Neutronics and Thermal Hydraulics Coupling Analysis on Novel Organic Cooled Reactor Based on Single-Channel Model ”. Fang et al. have contributed an article: “ Numerical Study on Heat Transfer and Enhancement Mechanism in PCM-Filled Shell-and-Tube Heat Exchangers ”. Su et al. have contributed an article entitled: “ Development and Assessment of an Isotropic Four-Equation Model for Heat Transfer of Low Prandtl Number Fluids ”.
Through revision and update for almost 1 year, this topic finally closed with above 14 papers published in the journal Frontiers in Energy Research. This topic presents a chance to public to follow these high-quality papers selected from this conference. The journal Frontiers in Energy Research will always open to accept more papers from international conferences. All the editors are welcomed to contact the journal for further information.
Author contributions
LZ is the leading author. LP is the corresponding author. JW and WD contribute the review. All authors approved the submitted version.
Conflict of interest
The authors declare that the research was conducted in the absence of any commercial or financial relationships that could be construed as a potential conflict of interest.
Publisher’s note
All claims expressed in this article are solely those of the authors and do not necessarily represent those of their affiliated organizations, or those of the publisher, the editors and the reviewers. Any product that may be evaluated in this article, or claim that may be made by its manufacturer, is not guaranteed or endorsed by the publisher.
Keywords: nuclear reactor, thermal, hydraulics, experiments, simulation
Citation: Zhang L, Pan L, Wang J and Ding W (2022) Editorial: Experimental and simulation research on nuclear reactor thermal-hydraulics. Front. Energy Res. 10:1028698. doi: 10.3389/fenrg.2022.1028698
Received: 26 August 2022; Accepted: 06 September 2022; Published: 23 September 2022.
Edited and reviewed by:
Copyright © 2022 Zhang, Pan, Wang and Ding. This is an open-access article distributed under the terms of the Creative Commons Attribution License (CC BY). The use, distribution or reproduction in other forums is permitted, provided the original author(s) and the copyright owner(s) are credited and that the original publication in this journal is cited, in accordance with accepted academic practice. No use, distribution or reproduction is permitted which does not comply with these terms.
*Correspondence: Luteng Zhang, [email protected] ; Liangming Pan, [email protected]
This article is part of the Research Topic
Experimental and Simulation Research on Nuclear Reactor Thermal-Hydraulics

An official website of the United States government
The .gov means it’s official. Federal government websites often end in .gov or .mil. Before sharing sensitive information, make sure you’re on a federal government site.
The site is secure. The https:// ensures that you are connecting to the official website and that any information you provide is encrypted and transmitted securely.
- Publications
- Account settings
Preview improvements coming to the PMC website in October 2024. Learn More or Try it out now .
- Advanced Search
- Journal List
- v.45(Suppl 1); 2016 Jan

Nuclear power in the 21st century: Challenges and possibilities
Akos horvath.
MTA Centre for Energy Research, KFKI Campus, P.O.B. 49, Budapest 114, 1525 Hungary
Elisabeth Rachlew
Department of Physics, Royal Institute of Technology, KTH, 10691 Stockholm, Sweden
The current situation and possible future developments for nuclear power—including fission and fusion processes—is presented. The fission nuclear power continues to be an essential part of the low-carbon electricity generation in the world for decades to come. There are breakthrough possibilities in the development of new generation nuclear reactors where the life-time of the nuclear waste can be reduced to some hundreds of years instead of the present time-scales of hundred thousand of years. Research on the fourth generation reactors is needed for the realisation of this development. For the fast nuclear reactors, a substantial research and development effort is required in many fields—from material sciences to safety demonstration—to attain the envisaged goals. Fusion provides a long-term vision for an efficient energy production. The fusion option for a nuclear reactor for efficient production of electricity has been set out in a focussed European programme including the international project of ITER after which a fusion electricity DEMO reactor is envisaged.
Introduction
All countries have a common interest in securing sustainable, low-cost energy supplies with minimal impact on the environment; therefore, many consider nuclear energy as part of their energy mix in fulfilling policy objectives. The discussion of the role of nuclear energy is especially topical for industrialised countries wishing to reduce carbon emissions below the current levels. The latest report from IPCC WGIII ( 2014 ) (see Box 1 for explanations of all acronyms in the article) says: “Nuclear energy is a mature low-GHG emission source of base load power, but its share of global electricity has been declining since 1993. Nuclear energy could make an increasing contribution to low-carbon energy supply, but a variety of barriers and risks exist ”.
Demand for electricity is likely to increase significantly in the future, as current fossil fuel uses are being substituted by processes using electricity. For example, the transport sector is likely to rely increasingly on electricity, whether in the form of fully electric or hybrid vehicles, either using battery power or synthetic hydrocarbon fuels. Here, nuclear power can also contribute, via generation of either electricity or process heat for the production of hydrogen or other fuels.
In Europe, in particular, the public opinion about safety and regulations with nuclear power has introduced much critical discussions about the continuation of nuclear power, and Germany has introduced the “Energiewende” with the goal to close all their nuclear power by 2022. The contribution of nuclear power to the electricity production in the different countries in Europe differs widely with some countries having zero contribution (e.g. Italy, Lithuania) and some with the major part comprising nuclear power (e.g. France, Hungary, Belgium, Slovakia, Sweden).
Current status
The use of nuclear energy for commercial electricity production began in the mid-1950s. In 2013, the world’s 392 GW of installed nuclear capacity accounted for 11 % of electricity generation produced by around 440 nuclear power plants situated in 30 countries (Fig. 1 ). This share has declined gradually since 1996, when it reached almost 18 %, as the rate of new nuclear additions (and generation) has been outpaced by the expansion of other technologies. After hydropower, nuclear is the world’s second-largest source of low-carbon electricity generation (IEA 2014 1 ).

Total number of operating nuclear reactors worldwide. The total number of reactors also include six in Taiwan (source: IAEA 2015) ( https://www.iaea.org/newscenter/focus/nuclear-power )
The Country Nuclear Power Profiles (CNPP 2 ) compiles background information on the status and development of nuclear power programmes in member states. The CNPP’s main objectives are to consolidate information about the nuclear power infrastructures in participating countries, and to present factors related to the effective planning, decision-making and implementation of nuclear power programmes that together lead to safe and economical operations of nuclear power plants.
Within the European Union, 27 % of electricity production (13 % of primary energy) is obtained from 132 nuclear power plants in January 2015 (Fig. 1 ). Across the world, 65 new reactors are under construction, mainly in Asia (China, South Korea, India), and also in Russia, Slovakia, France and Finland. Many other new reactors are in the planning stage, including for example, 12 in the UK.
Apart from one first Generation “Magnox” reactor still operating in the UK, the remainder of the operating fleet is of the second or third Generation type (Fig. 2 ). The predominant technology is the Light Water Reactor (LWR) developed originally in the United States by Westinghouse and then exploited massively by France and others in the 1970s as a response to the 1973 oil crisis. The UK followed a different path and pursued the Advanced Gas-cooled Reactor (AGR). Some countries (France, UK, Russia, Japan) built demonstration scale fast neutron reactors in the 1960s and 70s, but the only commercial reactor of this type currently operating is in Russia.

Nuclear reactor generations from the pioneering age to the next decade (reproduced with permission from Ricotti 2013 )
Future evolution
The fourth Generation reactors, offering the potential of much higher energy recovery and reduced volumes of radioactive waste, are under study in the framework of the “Generation IV International Forum” (GIF) 3 and the “International Project on Innovative Nuclear Reactors and Fuel Cycles” (INPRO). The European Commission in 2010 launched the European Sustainable Nuclear Industrial Initiative (ESNII), which will support three Generation IV fast reactor projects as part of the EU’s plan to promote low-carbon energy technologies. Other initiatives supporting biomass, wind, solar, electricity grids and carbon sequestration are in parallel. ESNII will take forward: the Astrid sodium-cooled fast reactor (SFR) proposed by France, the Allegro gas-cooled fast reactor (GFR) supported by central and eastern Europe and the MYRRHA lead- cooled fast reactor (LFR) technology pilot proposed by Belgium.
The generation of nuclear energy from uranium produces not only electricity but also spent fuel and high-level radioactive waste (HLW) as a by-product. For this HLW, a technical and socially acceptable solution is necessary. The time scale needed for the radiotoxicity of the spent fuel to drop to the level of natural uranium is very long (i.e. of the order of 200 000–300 000 years). The preferred solution for disposing of spent fuel or the HLW resulting from classical reprocessing is deep geological storage. Whilst there are no such geological repositories operating yet in the world, Sweden, Finland and France are on track to have such facilities ready by 2025 (Kautsky et al. 2013 ). In this context it should also be mentioned that it is only for a minor fraction of the HLW that recycling and transmutation is required since adequate separation techniques of the fuel can be recycled and again fed through the LWR system.
The “Strategic Energy Technology Plan” (SET-Plan) identifies fission energy as one of the contributors to the 2050 objectives of a low-carbon energy mix, relying on the Generation-3 reactors, closed fuel cycle and the start of implementation of Generation IV reactors making nuclear energy more sustainable. The EU Energy Roadmap 2050 provides decarbonisation scenarios with different assumptions from the nuclear perspective: two scenarios contemplate a nuclear phase-out by 2050, whilst three others consider that 15–20 % of electricity will be produced by nuclear energy. If by 2050 a generation capacity of 20 % nuclear electricity (140 GWe) is to be secured, 100–120 nuclear power units will have to be built between now and 2050, the precise number depending on the power rating (Garbil and Goethem 2013 ).
Despite the regional differences in the development plans, the main questions are of common interest to all countries, and require solutions in order to maintain nuclear power in the power mix of contributing to sustainable economic growth. The questions include (i) maintaining safe operation of the nuclear plants, (ii) securing the fuel supplies, (iii) a strategy for the management of radioactive waste and spent nuclear fuel.
Safety and non-proliferation risks are managed in accordance with the international rules issued both by IAEA and EURATOM in the EU. The nuclear countries have signed the corresponding agreements and the majority of them have created the necessary legal and regulatory structure (Nuclear Safety Authority). As regards radioactive wastes, particularly high-level wastes (HLW) and spent fuel (SF) most of the countries have long-term policies. The establishment of new nuclear units and the associated nuclear technology developments offer new perspectives, which may need reconsideration of fuel cycle policies and more active regional and global co-operation.
Open and closed fuel cycle
In the frame of the open fuel cycle, the spent fuel will be taken to final disposal without recycling. Deep geological repositories are the only available option for isolating the highly radioactive materials for a very long time from the biosphere. Long-term (80–100 years) near soil intermediate storages are realised in e.g. France and the Netherlands which will allow for permanent access and inspection. The main advantage of the open fuel cycle is its simplicity. The spent fuel assemblies are first stored in interim storage for several years or decades, then they will be placed in special containers and moved into deep underground storage facilities. The technology for producing such containers and for excavation of the underground system of tunnels exists today (Hózer et al. 2010 ; Kautsky et al. 2013 ).
The European Academies Science Advisory Board recently released the report on “Management of spent nuclear fuel and its waste” (EASAC 2014 ). The report discusses the challenges associated with different strategies to manage spent nuclear fuel, in respect of both open cycles and steps towards closing the nuclear fuel cycle. It integrates the conclusions on the issues raised on sustainability, safety, non-proliferation and security, economics, public involvement and on the decision-making process. Recently Vandenbosch et al. ( 2015 ) critically discussed the issue of confidence in the indefinite storage of nuclear waste. One complication of the nuclear waste storage problem is that the minor actinides represent a high activity (see Fig. 3 ) and pose non-proliferation issues to be handled safely in a civil used plant. This might be a difficult challenge if the storage is to be operated economically together with the fuel fabrication.

Radiotoxicity of radioactive waste
The open (or ‘once through’) cycle only uses part of the energy stored in the fuel, whilst effectively wasting substantial amounts of energy that could be recovered through recycling. The conventional closed fuel cycle strategy uses the reprocessing of the spent fuel following interim storage. The main components which can be further utilised (U and Pu) are recycled to fuel manufacturing (MOX (Mixed Oxide) fuel fabrication), whilst the smaller volume of residual waste in appropriately conditioned form—e.g. vitrified and encapsulated—is disposed of in deep geological repositories.
The advanced closed fuel cycle strategy is similar to the conventional one, but within this strategy the minor actinides are also removed during reprocessing. The separated isotopes are transmuted in combination with power generation and only the net reprocessing wastes and those conditioned wastes generated during transmutation will be, following appropriate encapsulation, disposed of in deep geological repositories. The main factor that determines the overall storage capacity of a long-term repository is the heat content of nuclear waste, not its volume. During the anticipated repository time, the specific heat generated during the decay of the stored HLW must always stay below a dedicated value prescribed by the storage concept and the geological host information. The waste that results from reprocessing spent fuel from thermal reactors has a lower heat content (after a period of cooling) than does the spent fuel itself. Thus, it can be stored more densely.
A modern light water reactor of 1 GWe capacity will typically discharge about 20–25 tonnes of irradiated fuel per year of operation. About 93–94 % of the mass of typical uranium oxide irradiated fuel comprises uranium (mostly 238 U), with about 4–5 % fission products and ~1 % plutonium. About 0.1–0.2 % of the mass comprises minor actinides (neptunium, americium and curium). These latter elements accumulate in nuclear fuel because of neutron capture, and they contribute significantly to decay heat loading and neutron output, as well as to the overall radiotoxic hazard of spent fuel. Although the total minor actinide mass is relatively small—20 to 25 kg per year from a 1 GWe LWR—it has a disproportionate impact on spent fuel disposal because of its long radioactive decay times (OECD Nuclear Energy Agency 2013 ).
Generation IV development
To address the issue of sustainability of nuclear energy, in particular the use of natural resources, fast neutron reactors (FNRs) must be developed, since they can typically multiply by over a factor 50 the energy production from a given amount of uranium fuel compared to current reactors. FNRs, just as today’s fleet, will be primarily dedicated to the generation of fossil-free base-load electricity. In the FNR the fuel conversion ratio (FCR) is optimised. Through hardening the spectrum a fast reactor can be designed to burn minor actinides giving a FCR larger than unity which allows breeding of fissile materials. FNRs have been operated in the past (especially the Sodium-cooled Fast Reactor in Europe), but today’s safety, operational and competitiveness standards require the design of a new generation of fast reactors. Important research and development is currently being coordinated at the international level through initiatives such as GIF.
In 2002, six reactor technologies were selected which GIF believe represent the future of nuclear energy. These were selected from the many various approaches being studied on the basis of being clean, safe and cost-effective means of meeting increased energy demands on a sustainable basis. Furthermore, they are considered being resistant to diversion of materials for weapons proliferation and secure from terrorist attacks. The continued research and development will focus on the chosen six reactor approaches. Most of the six systems employ a closed fuel cycle to maximise the resource base and minimise high-level wastes to be sent to a repository. Three of the six are fast neutron reactors (FNR) and one can be built as a fast reactor, one is described as epithermal, and only two operate with slow neutrons like today’s plants. Only one is cooled by light water, two are helium-cooled and the others have lead–bismuth, sodium or fluoride salt coolant. The latter three operate at low pressure, with significant safety advantage. The last has the uranium fuel dissolved in the circulating coolant. Temperatures range from 510 to 1000 °C, compared with less than 330 °C for today’s light water reactors, and this means that four of them can be used for thermochemical hydrogen production.
The sizes range from 150 to 1500 MWe, with the lead-cooled one optionally available as a 50–150 MWe “battery” with long core life (15–20 years without refuelling) as replaceable cassette or entire reactor module. This is designed for distributed generation or desalination. At least four of the systems have significant operating experience already in most respects of their design, which provides a good basis for further research and development and is likely to mean that they can be in commercial operation well before 2030. However, when addressing non-proliferation concerns it is significant that fast neutron reactors are not conventional fast breeders, i.e. they do not have a blanket assembly where plutonium-239 is produced. Instead, plutonium production happens to take place in the core, where burn-up is high and the proportion of plutonium isotopes other than Pu-239 remains high. In addition, new reprocessing technologies will enable the fuel to be recycled without separating the plutonium.
In January 2014, a new GIF Technology Roadmap Update was published. 4 It confirmed the choice of the six systems and focused on the most relevant developments of them so as to define the research and development goals for the next decade. It suggested that the Generation IV technologies most likely to be deployed first are the SFR, the lead-cooled fast reactor (LFR) and the very high temperature reactor technologies. The molten salt reactor and the GFR were shown as furthest from demonstration phase.
Europe, through sustainable nuclear energy technology platform (SNETP) and ESNII, has defined its own strategy and priorities for FNRs with the goal to demonstrate Generation IV reactor technologies that can close the nuclear fuel cycle, provide long-term waste management solutions and expand the applications of nuclear fission beyond electricity production to hydrogen production, industrial heat and desalination; The SFR as a proven concept, as well as the LFR as a short-medium term alternative and the GFR as a longer-term alternative technology. The French Commissariat à l’Energie Atomique (CEA) has chosen the development of the SFR technology. Astrid (Advanced Sodium Technological Reactor for Industrial Demonstration) is based on about 45 reactor-years of operational experience in France and will be rated 250 to 600 MWe. It is expected to be built at Marcoule from 2017, with the unit being connected to the grid in 2022.
Other countries like Belgium, Italy, Sweden and Romania are focussing their research and development effort on the LFR whereas Hungary, Czech Republic and Slovakia are investing in the research and development on GFR building upon the work initiated in France on GFR as an alternative technology to SFR. Allegro GFR is to be built in eastern Europe, and is more innovative. It is rated at 100 MWt and would lead to a larger industrial demonstration unit called GoFastR. The Czech Republic, Hungary and Slovakia are making a joint proposal to host the project, with French CEA support. Allegro is expected to begin construction in 2018 operate from 2025. The industrial demonstrator would follow it.
In mid-2013, four nuclear research institutes and engineering companies from central Europe’s Visegrád Group of Nations (V4) agreed to establish a centre for joint research, development and innovation in Generation IV nuclear reactors (the Czech Republic, Hungary, Poland and Slovakia) which is focused on gas-cooled fast reactors such as Allegro.
The MYRRHA (Multi-purpose hYbrid Research Reactor for High-tech Applications) 5 project proposed in Belgium by SCK•CEN could be an Experimental Technological Pilot Plant (ETPP) for the LFR technology. Later, it could become a European fast neutron technology pilot plant for lead and a multi-purpose research reactor. The unit is rated at 100 thermal MW and has started construction at SCK-CEN’s Mol site in 2014 planned to begin operation in 2023. A reduced-power model of Myrrha called Guinevere started up at Mol in March 2010. ESNII also includes an LFR technology demonstrator known as Alfred, also about 100 MWt, seen as a prelude to an industrial demonstration unit of about 600 MWe. Construction on Alfred could begin in 2017 and the unit could start operating in 2025.
Research and development topics to meet the top-level criteria established within the GIF forum in the context of simultaneously matching economics as well as stricter safety criteria set-up by the WENRA FNR demand substantial improvements with respect to the following issues:
- Primary system design simplification,
- Improved materials,
- Innovative heat exchangers and power conversion systems,
- Advanced instrumentation, in-service inspection systems,
- Enhanced safety,
and those for fuel cycle issues pertain to:
- Partitioning and transmutation,
- Innovative fuels (including minor actinide-bearing) and core performance,
- Advanced separation both via aqueous processes supplementing the PUREX process as well as pyroprocessing, which is mandatory for the reprocessing of the high MA-containing fuels,
- Develop a final depository.
Beyond the research and development, the demonstration projects mentioned above are planned in the frame of the SET-Plan ESNII for sustainable fission. In addition, supporting research infrastructures, irradiation facilities, experimental loops and fuel fabrication facilities, will need to be constructed.
Regarding transmutation, the accelerator-driven transmutation systems (ADS) technology must be compared to FNR technology from the point of view of feasibility, transmutation efficiency and cost efficiency. It is the objective of the MYRRHA project to be an experimental demonstrator of ADS technology. From the economical point of view, the ADS industrial solution should be assessed in terms of its contribution to closing the fuel cycle. One point of utmost importance for the ADS is its ability for burning larger amounts of minor actinides (the typical maximum in a critical FNR is about 2 %).
The concept of partitioning and transmutation (P&T) has three main goals: reduce the radiological hazard associated with spent fuel by reducing the inventory of minor actinides, reduce the time interval required to reach the radiotoxicity of natural uranium and reduce the heat load of the HLW packages to be stored in the geological disposal hence reducing the foot print of the geological disposal.
Advanced management of HLW through P&T consists in advanced separation of the minor actinides (americium, curium and neptunium) and some fission products with a long half-life present in the nuclear waste and their transmutation in dedicated burners to reduce the radiological and heat loads on the geological disposal. The time scale needed for the radiotoxicity of the waste to drop to the level of natural uranium will be reduced from a ‘geological’ value (300 000 years) to a value that is comparable to that of human activities (few hundreds of years) (OECD/NEA 2006 ; OECD 2012 ; PATEROS 2008 6 ). Transmutation of the minor actinides is achieved through fission reactions and therefore fast neutrons are preferred in dedicated burners.
At the European level, four building blocks strategy for Partitioning and Transmutation have been identified. Each block poses a serious challenge in terms of research & development to be done in order to reach industrial scale deployment. These blocks are:
- Demonstration of advanced reprocessing of spent nuclear fuel from LWRs, separating Uranium, Plutonium and Minor Actinides;
- Demonstration of the capability to fabricate at semi-industrial level dedicated transmuter fuel heavily loaded in minor actinides;
- Design and construct one or more dedicated transmuters;
- Fabrication of new transmuter fuel together with demonstration of advanced reprocessing of transmuter fuel.
MYRRHA will support this Roadmap by playing the role of an ADS prototype (at reasonable power level) and as a flexible irradiation facility providing fast neutrons for the qualification of materials and fuel for an industrial transmuter. MYRRHA will be not only capable of irradiating samples of such inert matrix fuels but also of housing fuel pins or even a limited number of fuel assemblies heavily loaded with MAs for irradiation and qualification purposes.
Options for nuclear fusion beyond 2050
Nuclear fusion research, on the basis of magnetic confinement, considered in this report, has been actively pursued in Europe from the mid-60s. Fusion research has the goal to achieve a clean and sustainable energy source for many generations to come. In parallel with basic high-temperature plasma research, the fusion technology programme is pursued as well as the economy of a future fusion reactor (Ward et al. 2005 ; Ward 2009 ; Bradshaw et al. 2011 ). The goal-oriented fusion research should be driven with an increased effort to be able to give the long searched answer to the open question, “will fusion energy be able to cover a major part of mankind’s electricity demand?”. ITER, the first fusion reactor to be built in France by the seven collaborating partners (Europe, USA, Russia, Japan, Korea, China, India) is hoped to answer most of the open physics and many of the remaining technology/material questions. ITER is expected to start operation of the first plasma around 2020 and D-T operation 2027.
The European fusion research has been successful through the organisation of EURATOM to which most countries in Europe belong (the fission programme is also included in EURATOM). EUROfusion, the European Consortium for the Development of Fusion Energy, manages European fusion research activities on behalf of EURATOM. The organisation of the research has resulted in a well-focused common fusion research programme. The members of the EUROfusion 7 consortium are 29 national fusion laboratories. EUROfusion funds all fusion research activities in accordance with the “EFDA Fusion electricity. Roadmap to the realisation of fusion energy” (EFDA 2012 , Fusion electricity). The Roadmap outlines the most efficient way to realise fusion electricity. It is the result of an analysis of the European Fusion Programme undertaken by all Research Units within EUROfusion’s predecessor agreement, the European Fusion Development Agreement, EFDA.
The most successful confinement concepts are toroidal ones like tokamaks and helical systems like stellarators (Wagner 2012 , 2013 ). To avoid drift losses, two magnetic field components are necessary for confinement and stability—the toroidal and the poloidal field component. Due to their superposition, the magnetic field winds helically around a system of nested toroids. In both cases, tokamak and stellarator, the toroidal field is produced by external coils; the poloidal field arises from a strong toroidal plasma current in tokamaks. In case of helical systems all necessary fields are produced externally by coils which have to be superconductive when steady-state operation is intended. Europe is constructing the most ambitious stellarator, Wendelstein 7-X in Germany. It is a fully optimised system with promising features. W7-X goes into operation in 2015. 8
Fusion research has now reached plasma parameters needed for a fusion reactor, even if not all parameters are reached simultaneously in a single plasma discharge (see Fig. 4 ). Plotted is the triple product n•τ E• T i composed of the density n, the confinement time τ E and the ion temperature T i . For ignition of a deuterium–tritium plasma, when the internal α-particle heating from the DT-reaction takes over and allows the external heating to be switched off, the triple product has to be about >6 × 10 21 m −3 s keV). The record parameters given as of today are shown together with the fusion experiment of its achievement in Fig. 4 . The achieved parameters and the missing factors to the ultimate goal of a fusion reactor are summarised below:
- Temperature: 40 keV achieved (JT-60U, Japan); the goal is surpassed by a factor of two
- Density n surpassed by factor 5 (C-mod,USA; LHD,Japan)
- Energy confinement time: a factor of 4 is missing (JET, Europe)
- Fusion triple product (see Fig. 4 : a factor of 6 is missing (JET, Europe)
- The first scientific goal is achieved: Q (fusion power/external heating power) ~1 (0,65) (JET, Europe)
- D-T operation without problems (TFTR (USA), JET, small tritium quantities have been used, however)
- Maximal fusion power for short pulse: 16 MW (JET)
- Divertor development (ASDEX, ASDEX-Upgrade, Germany)
- Design for the first experimental reactor complete (ITER, see below)
- The optimisation of stellarators (W7-AS, W7-X, Germany)

Progress in fusion parameters. Derived in 1955, the Lawson criterion specifies the conditions that must be met for fusion to produce a net energy output (1 keV × 12 million K). From this, a fusion “triple product” can be derived, which is defined as the product of the plasma ion density, ion temperature and energy confinement time. This product must be greater than about 6 × 10 21 keV m −3 s for a deuterium–tritium plasma to ignite. Due to the radioactivity associated with tritium, today’s research tokamaks generally operate with deuterium only ( solid dots ). The large tokamaks JET(EU) and TFTR(US), however, have used a deuterium–tritium mix ( open dots ). The rate of increase in tokamak performance has outstripped that of Moore’s law for the miniaturisation of silicon chips (Pitts et al. 2006 ). Many international projects (their names are given by acronyms in the figure) have contributed to the development of fusion plasma parameters and the progress in fusion research which serves as the basis for the ITER design
After 50 years of fusion research there is no evidence for a fundamental obstacle in the basic physics. But still many problems have to be overcome as detailed below:
Critical issues in fusion plasma physics based on magnetic confinement
- confine a plasma magnetically with 1000 m 3 volume,
- maintain the plasma stable at 2–4 bar pressure,
- achieve 15 MA current running in a fluid (in case of tokamaks, avoid instabilities leading to disruptions),
- find methods to maintain the plasma current in steady-state,
- tame plasma turbulence to get the necessary confinement time,
- develop an exhaust system (divertor) to control power and particle exhaust, specifically to remove the α-particle heat deposited into the plasma and to control He as the fusion ash.
Critical issues in fusion plasma technology
- build a system with 200 MKelvin in the plasma core and 4 Kelvin about 2 m away,
- build magnetic system at 6 Tesla (max field 12 Tesla) with 50 GJ energy,
- develop heating systems to heat the plasma to the fusion temperature and current drive systems to maintain steady-state conditions for the tokamak,
- handle neutron-fluxes of 2 MW/m 2 leading to 100 dpa in the surrounding material,
- develop low activation materials,
- develop tritium breeding technologies,
- provide high availability of a complex system using an appropriate remote handling system,
- develop the complete physics and engineering basis for system licensing.
The goals of ITER
The major goals of ITER (see Fig. 5 ) in physics are to confine a D-T plasma with α-particle self-heating dominating all other forms of plasma heating, to produce about ~500 MW of fusion power at a gain Q = fusion power/external heating power, of about 10, to explore plasma stability in the presence of energetic α-particles, and to demonstrate ash-exhaust and burn control.

Schematic layout of the ITER reactor experiment (from www.iter.org )
In the field of technology, ITER will demonstrate fundamental aspects of fusion as the self-heating of the plasma by alpha-particles, show the essentials to a fusion reactor in an integrated system, give the first test a breeding blanket and assess the technology and its efficiency, breed tritium from lithium utilising the D-T fusion neutron, develop scenarios and materials with low T-inventories. Thus ITER will provide strong indications for vital research and development efforts necessary in the view of a demonstration reactor (DEMO). ITER will be based on conventional steel as structural material. Its inner wall will be covered with beryllium to surround the plasma with low-Z metal with low inventory properties. The divertor will be mostly from tungsten to sustain the high α-particle heat fluxes directed onto target plates situated inside a divertor chamber. An important step in fusion reactor development is the achievement of licensing of the complete system.
The rewards from fusion research and the realisation of a fusion reactor can be described in the following points:
- fusion has a tremendous potential thanks to the availability of deuterium and lithium as primary fuels. But as a recommendation, the fusion development has to be accelerated,

Fusion time strategy towards the fusion reactor on the net (EFDA 2012 , Fusion electricity. A roadmap to the realisation of fusion energy)
In addition, there is the fusion technology programme and its material branch, which ultimately need a neutron source to study the interaction with 14 MeV neutrons. For this purpose, a spallation source IFMIF is presently under design. As a recommendation, ways have to be found to accelerate the fusion development. In general, with ITER, IFMIF and the DEMO, the programme will move away from plasma science more towards technology orientation. After the ITER physics and technology programme—if successful—fusion can be placed into national energy supply strategies. With fusion, future generations can have access to a clean, safe and (at least expected of today) economic power source.
The fission nuclear power continues to be an essential part of the low-carbon electricity generation in the world for decades to come. There are breakthrough possibilities in the development of new generation nuclear reactors where the life-time of the nuclear waste can be reduced to some hundreds of years instead of the present time-scales of hundred thousand of years. Research on the fourth generation reactors is needed for the realisation of this development. For the fast nuclear reactors a substantial research and development effort is required in many fields—from material sciences to safety demonstration—to attain the envisaged goals. Fusion provides a long-term vision for an efficient energy production. The fusion option for a nuclear reactor for efficient production of electricity should be vigorously pursued on the international arena as well as within the European energy roadmap to reach a decision point which allows to critically assess this energy option.
Box 1 Explanations of abbreviations used in this article
Biographies.
is Professor in Energy Research and Director of MTA Center for Energy Research, Budapest, Hungary. His research interests are in the development of new fission reactors, new structural materials, high temperature irradiation resistance, mechanical deformation.
is Professor of Applied Atomic and Molecular Physics at Royal Institute of Technology, (KTH), Stockholm, Sweden. Her research interests are in basic atomic and molecular processes studied with synchrotron radiation, development of diagnostic techniques for analysing the performance of fusion experiments in particular development of photon spectroscopic diagnostics.
1 http://www.iea.org/ .
2 https://cnpp.iaea.org/pages/index.htm .
3 GenIV International forum: ( http://www.gen-4.org/index.html ).
4 https://www.gen-4.org/gif/jcms/c_60729/technology-roadmap-update-2013 .
5 http://myrrha.sckcen.be/ .
6 www.sckcen.be/pateros/ .
7 https://www.euro-fusion.org/ .
8 https://www.ipp.mpg.de/ippcms/de/pr/forschung/w7x/index.html .
Contributor Information
Akos Horvath, Email: [email protected] .
Elisabeth Rachlew, Email: es.htk@kre .
- Bradshaw AM, Hamacher T, Fischer U. Is nuclear fusion a sustainable energy form? Fusion Engineering and Design. 2011; 86 :2770–2773. doi: 10.1016/j.fusengdes.2010.11.040. [ CrossRef ] [ Google Scholar ]
- EASAC. 2014. EASAC Report 23—Management of spent nuclear fuel and its waste. http://www.easac.eu/energy/reports-and-statements/detail-view/article/management-o.html .
- EFDA. 2012. Fusion electricity. A roadmap to the realization of fusion energy. https://www.euro-fusion.org/wpcms/wp-content/uploads/2013/01/JG12.356-web.pdf .
- Garbil, R., and G. Van Goethem. (ed.). 2013. Symposium on the “Benefits and limitations of nuclear fission for a low carbon economy”, European Commission, Brussels, ISBN 978-92.79.29833.2.
- Hózer, Z. S. Borovitskiy, G. Buday, B. Boullis, G. Cognet, S. A. Delichatsios, J. Gadó, A. Grishin, et al. 2010. Regional strategies concerning nuclear fuel cycle and HLRW in Central and Eastern European Countries. International conference on management of spent fuel from Nuclear Power Reactors, Vienna, Conference ID:38089 (CN-178).
- IEA (International Energy Authority). 2014. World Energy Outlook 2014. http://www.iea.org/ .
- IPCC. 2014. Summary for policymakers WGIII AR5, SPM.4.2.2 Energy supply.
- Kautsky, U., T. Lindborg, and J. Valentin (ed.). 2013. Humans and ecosystems over the coming millenia: A biosphere assessment of radioactive waste disposal in Sweden. Ambio 42(4): 381–526. [ PMC free article ] [ PubMed ]
- OECD. 2011–2012. Fact book: Economic, environmental and social statistics. Retrieved from http://www.oecd-ilibrary.org/economics/oecd-factbook-2011-2012_factbook-2011-en .
- OECD/NEA. 2006. Potential benefits and impacts of advanced nuclear fuel cycles with actinide partitioning and transmutation. ISBN: 978-92-64-99165-1, http://www.oecd-nea.org/science/reports/2011/6894-benefits-impacts-advanced-fuel.pdf .
- OECD Nuclear Energy Agency. 2013. Minor actinide burning in thermal reactors. A report by the Working Party on Scientific Issues of Reactor Systems, NEA #6997. http://www.oecd-nea.org/science/pubs/2013/6997-minor-actinide.pdf .
- Pitts R, Buttery R, Pinches S. Fusion: The way ahead. Physics World. 2006; 19 :20–26. doi: 10.1088/2058-7058/19/3/35. [ CrossRef ] [ Google Scholar ]
- Ricotti ME. Nuclear energy: Basics, present, future. EPJ Web of Conferences. 2013; 54 :01005. doi: 10.1051/epjconf/20135401005. [ CrossRef ] [ Google Scholar ]
- Vandenbosch R, Vandenbosch SE. Nuclear waste confidence: Is indefinite storage safe? APS Physics and Society. 2015; 44 :5–7. [ Google Scholar ]
- Wagner, F. 2012. Fusion energy by magnetic confinement. IPP 18/3, http://hdl.handle.net/11858/00-001M-0000-0026-E767-A .
- Wagner F. Physics of magnetic confinement fusion. EPJ Web of Conferences. 2013; 54 :01007. doi: 10.1051/epjconf/20135401007. [ CrossRef ] [ Google Scholar ]
- Ward DJ. The contribution of fusion to sustainable development. Fusion Engineering and Design. 2009; 82 :528–533. doi: 10.1016/j.fusengdes.2007.02.028. [ CrossRef ] [ Google Scholar ]
- Ward DJ, Cook I, Lechon Y, Saez R. The economic viability of fusion power. Engineering and Design. 2005; 75–79 :1221–1227. doi: 10.1016/j.fusengdes.2005.06.160. [ CrossRef ] [ Google Scholar ]
Advertisement
India’s nuclear power program: a critical review
- Published: 30 August 2022
- Volume 47 , article number 181 , ( 2022 )
Cite this article
- G Vaidyanathan ORCID: orcid.org/0000-0002-6549-4377 1 &
- R D Kale 2
375 Accesses
2 Citations
3 Altmetric
Explore all metrics
Global carbon emissions have been rising sharply since the start of the 20th century, and countries have adopted various policies in recent years to reduce greenhouse gas (GHG) emissions in different sectors. Nuclear energy is one energy source that is least polluting with minimum GHG emissions. India’s nuclear power programme started with Heavy water reactors in the first stage followed by Fast Reactors in the second stage. Third stage of Thorium utilisation is yet to start. The deployment of Pu/depleted U from Heavy water reactors in fast reactors would help in the effective utilisation of the indigenous uranium resources to a large extent besides reducing the waste. The thorium technology to obtain uranium 233 is equally important as India possesses large amounts of thorium deposits. With sufficient U233 we can provide a significant long-term solution to fuel our nuclear reactors to produce electricity needed for its development. Linked to the nuclear programme is the availability of fuel, ability to reprocess the spent fuel and manage the wastes. India’s waiver from the Nuclear Suppliers’ Group and its agreement with the global atomic energy body, IAEA, have resulted in limited breakthroughs in the nuclear sector in the last decade and allowed the import of fuel. This paper undertakes a review of the different steps taken by India in the nuclear arena and makes a realistic assessment of its current nuclear power programme.
This is a preview of subscription content, log in via an institution to check access.
Access this article
Price includes VAT (Russian Federation)
Instant access to the full article PDF.
Rent this article via DeepDyve
Institutional subscriptions
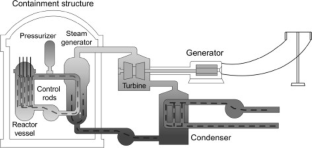
Abbreviations
Greenhouse emissions
International Atomic Energy Agency
Department of Atomic Energy India
Pressurised heavy water reactor
Rajasthan Atomic Power Station
Pressurised water reactor
Boiling water reactor
Sodium fast reactor
Canada Deutrium
Reaktor Bolshoy Moshchnosty Kanalny
Gas cooled reactor
Fast breeder reactor
Vodo-Vodyanoi Energetichesky Reaktor
Experimental breeder reactor I
Atomic Energy Commission
Atomic Energy Establishment, Trombay
Bhabha Atomic Research Centre
Fast breeder test reactor
Atomic Energy of Canada Limited
Peaceful nuclear explosion
Mixed oxide
Madras Atomic Power Station
Nuclear fuel complex
Kakrapar Atomic Power Station
Narora Atomic Power Station
Tarapur Atomic Power Station
Nuclear Power Corporation of India
Kalpakkam mini reactor
Prototype fast breeder reactor
Compact reprocessing of advanced fuel
Fast reactor fuel cycle facility
Molten salt reactor
Advanced heavy water reactor
Evolutionary power reactor
Atomic Energy Regulatory Board
Ministry of Environment and Forests
Interest during construction
Iyer M R 2020 The Saga of Atomic Energy in India- Why is Nuclear Power still Subcritical? Authors Press, Delhi
Google Scholar
Sarma N and Banerjee B 2008 Nuclear Power in India: A Critical History. Rupa & Co., Delhi
Hart D 1983 Nuclear Power in India: A Comparative Analysis. George Allen & Unwin, London. https://doi.org/10.4324/9780429278303
Book Google Scholar
Bhardwaj SA 2013 Indian nuclear power programme—Past, present, and future , S ̄adhan ̄a 38: Part 5, pp. 775–794
Kale R D 2020 India’s fast reactor programme—A review and critical assessment. Progress Nuclear Energy 122: 103265
Article Google Scholar
Vaidyanathan G and Purniah B 2020 Energy, and Environment- from Cradle to Grave. Yes Dee Publishing, Chennai
Grover RB 2006 Role of Nuclear Energy in India’s Energy Mix, IANCAS Bulletin , April
Uwe R Fritsche et al ., Energy and Land Use, September 2017, International renewable energy Agency IRENA& UN Convention to Combat, desertification, http://iinas.org/tl_files/iinas/downloads/land/IINAS_2017_UNCCD-IRENA_Energy-Land_paper.pdf
Neumeyer C and Goldston R 2016 Dynamic EROI Assessment of the IPCC 21st Century Electricity Production Scenario Sustainability 2016, 8, 421. https://doi.org/10.3390/su8050421
IAEA-TECDOC-892, Comparison of Energy Sources in Terms of Their Full-Energy-Chain Emission Factors of Greenhouse Gases, IAEA, Vienna, 1996
Nuclear Electricity Generation: What Are the External Costs? OECD, NEA4372, Nuclear Energy Agency, 2003. https://www.oecd-nea.org/jcms/pl_13756/nuclear-electricity-generation-what-are-the-external-costs?details=true
IAEA 2006 Nuclear Power Reactors in the World, International Atomic Energy Agency (IAEA). Austria, Vienna
Balachandran G and Kapil Patil K 2012 Nuclear Power Plant and Civil Nuclear Liability , Institute for Defence Studies and Analysis, Delhi
Shah JC et al. 1977 Experience from the Construction and Operation of Tarapur and Rajasthan Nuclear Power Station" [IAEA-CN-36/360 (VII.3)], Salzburg Conference
Sundaram CV, Krishnan LV and Iyengar TS 1998 Atomic energy in India: 50 years. Department of Atomic Energy, New Delhi
Agreement for cooperation between the Government of India and the Government of United States of America concerning peaceful uses of nuclear energy (2007). http://www.meaindia.nic.in/pressrelease/2007/08/03pr01.pdf
Sarma MSR, Sah BML and Subbaratnam T 1981 Dousing incident at RAPS Unit I, Regional seminar for Asia and the Pacific Region—radiation emergency preparedness (health physics and medical aspects), Kalpakkam, India, 30 Nov 1981
Sinha RK et al 2010 Plant life management (PLIM) practices for pressurised heavy water nuclear reactors (PHWR) in understanding and mitigating ageing in nuclear power plants Materials and operational aspects of plant life management (PLIM), Edited by Philip G. Tipping, Wood Head Publishing, pp. 739–794
Grover R B and Srinivasan M R 2020 Vikram Sarabhai: his vision for the development of atomic energy in India. Curr. Sci. 118(8): 1191–1195
Bharadwaj A, Krishnan LV and Rajagopal S 2017 Nuclear viewpoint in India, MRS energy & sustainability: a review. J. Mater. Res. Soc. , 4, Article number: 6
Sharma S and Mukherjee SP 2009 A Dream Comes True- The Saga of Heavy Water Production in India , Heavy Water Board, Mumbai
Bhasin V, Kushwaha HS and Kakodkar A 2011 Failure Analysis of MAPS Inlet Manifold. In Transactions of the 11th International Conference on Structural Mechanics in Reactor Technology, Tokyo
Kakodkar A 2019 Fire and Fury Rupa publications, 2019, P49 & 53
Sundarrajan AR, Parthasarathy KS and Sinha S 2008 Atomic Energy Regulatory Board- 25 years of Safety Regulation . https://www.aerb.gov.in/images/PDF/Silver_Jubilee_Book/SJBook.pdf0
Murugan S et al . 2013 Irradiation testing of zirconium alloys and stainless steel in fast breeder test reactor, India. In Effects of Radiation on Nuclear Materials : 25th Volume, ed. T. Yamamoto, M. Sokolov, and B. Hanson (West Conshohocken, PA: ASTM International), pp. 176–191
www.npcil.co.in
https://www.iaea.org/resources/databases/power-reactor-information-system-pris
Basu P C and Gupchup V N 2004 Safety evaluation of rehabilitation of delaminated containment dome. Nucl. Eng. Des. 228: 195–205
Rajamani N 2017 Reprocessing of spent nuclear fuel in India: present challenges and future programme. Prog. Nucl. Energy 101: 118
Manoharan N, Suresh Kumar KV and Srinivasan G 2011 Fifteen years of operating experience of Kamini Reactor , IAEA
Tongia R and Arunachalam V S 1998 India’s nuclear breeders: Technology, viability and options. Curr. Sci. 75(6): 549
RaoKR2001, Radioactive waste: the problem and its management. Curr. Sci. 81(12): 25
www.nuclear-power.net
Nagarajan K et al . 2011 Development of pyrochemical reprocessing for spent metal fuels. Energy Procedia 7: 431
Jess CG 2015 History of the ORNL molten salt program. In Workshop on Molten Salt Reactor Technologies , Oak Ridge National Laboratory
Paranjpe SR 1998 Fast Breeder Reactor Development in India (unpublished)
Vijayan P K 2015 Conceptual design of Indian molten salt breeder reactor. Pramana J. Phys. 85(3): 539
Nayak AK et al . 2019 Thorium technology development in an Indian perspective. In Thorium—Energy for the Future , A. K. Nayak and B. R. Sehgal (eds.), Springer Nature
Kakodkar A et al . 2019 Nuclear Power: India’s Development Imperative, Report Vivekananda International Foundation, Delhi
Grover R B 2017 The civil liability for nuclear damage act of India: An engineering perspective regarding Supplier’s liability. Prog. Nucl. Energy 101: 168
Grover R B and Puri R R 2013 Development of human resources for Indian nuclear power programme. Sadhana 38(5): 1051–1064
Bajaj S S 2013 Regulatory practices for nuclear power plants in India. Sadhana 38(5): 1027–1050
Mishra L 1999 India's Quest for Technological Self- Reliance , PhD Thesis, Jawaharlal Nehru University, Delhi
Lok Sabha Secretariat 1994 Ninth Report Standing Committee on Energy, Department of Atomic Energy Demands for Grants (1994-95) Presented to Parliament
Download references

Author information
Authors and affiliations.
Indira Gandhi Centre for Atomic Research (Formerly), Chennai, Tamil Nadu, 603210, India
G Vaidyanathan
Indira Gandhi Centre for Atomic Research (Formerly), Pune, Maharashtra, 411038, India
You can also search for this author in PubMed Google Scholar
Corresponding author
Correspondence to G Vaidyanathan .
Rights and permissions
Reprints and permissions
About this article
Vaidyanathan, G., Kale, R.D. India’s nuclear power program: a critical review. Sādhanā 47 , 181 (2022). https://doi.org/10.1007/s12046-022-01953-9
Download citation
Received : 23 March 2021
Revised : 02 November 2021
Accepted : 13 July 2022
Published : 30 August 2022
DOI : https://doi.org/10.1007/s12046-022-01953-9
Share this article
Anyone you share the following link with will be able to read this content:
Sorry, a shareable link is not currently available for this article.
Provided by the Springer Nature SharedIt content-sharing initiative
- construction
- Find a journal
- Publish with us
- Track your research
share this!
March 19, 2024
This article has been reviewed according to Science X's editorial process and policies . Editors have highlighted the following attributes while ensuring the content's credibility:
fact-checked
trusted source
written by researcher(s)
How do we tell future generations about highly radioactive nuclear waste repositories?
by Thomas Keating and Anna Storm, The Conversation
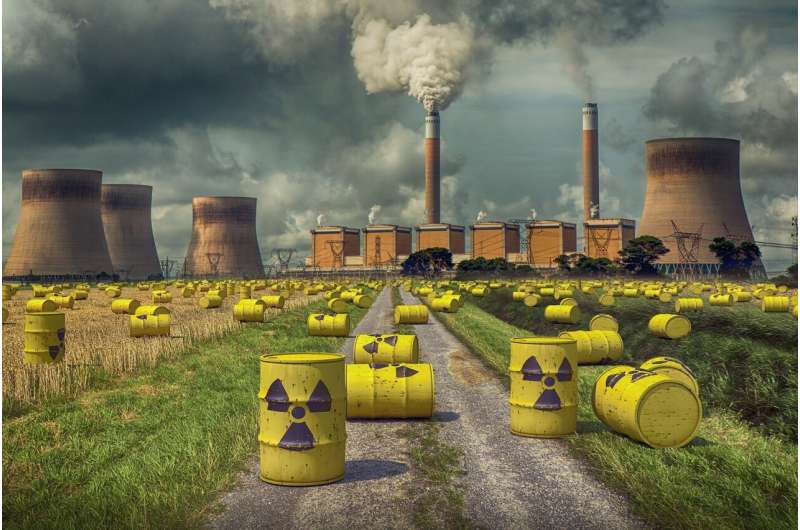
In Europe, increasing efforts on climate change mitigation, a sudden focus on energy independence after Russia's invasion of Ukraine, and reported breakthroughs in nuclear fusion have sparked renewed interest in the potential of nuclear power. So-called small modular reactors (SMRs) are increasingly under development, and familiar promises about nuclear power's potential are being revived.
Nuclear power is routinely portrayed by proponents as the source of "limitless" amounts of carbon-free electricity. The rhetorical move from speaking about " renewable energy " to "fossil-free energy" is increasingly evident, and telling.
Yet nuclear energy production requires managing what is known as "spent" nuclear fuel where major problems arise about how best to safeguard these waste materials into the future—especially should nuclear energy production increase. Short-term storage facilities have been in place for decades, but the question of their long-term deposition has caused intense political debates , with a number of projects being delayed or cancelled entirely . In the United States, work on the Yucca Mountain facility has stopped completely leaving the country with 93 nuclear reactors and no long-term storage site for the waste they produce.
Nuclear power plants produce three kinds of radioactive waste :
- Short-lived low- and intermediate-level waste;
- Long-lived low- and intermediate-level waste;
- Long-lived and highly radioactive waste, known as spent nuclear fuel.
The critical challenge for nuclear energy production is the management of long-lived waste, which refers to nuclear materials that take thousands of years to return to a level of radioactivity that is deemed "safe". According to the US Nuclear Regulatory Commission (NRC), in spent fuel half of the radiation in strontium-90 and cesium-137 can decay in 30 years, while it would take 24,000 years for plutonium-239 to return to a state considered "harmless." However, exactly what is meant by "safe" and "harmless" in this context is something that remains poorly defined by international nuclear management organizations, and there is surprisingly little international consensus about the time it takes for radioactive waste to return to a state considered "safe" for organic life.
'Permanent' geological repositories
Despite the seeming revival of nuclear energy production today, very few of the countries that produce nuclear energy have defined a long-term strategy for managing highly radioactive spent fuel into the future. Only Finland and Sweden have confirmed plans for so-called "final" or "permanent" geological repositories.
The Swedish government granted approval for a final repository in the village of Forsmark in January 2022, with plans to construct, fill and seal the facility over the next century. This repository is designed to last 100,000 years, which is how long planners say that it will take to return to a level of radioactivity comparable to uranium found in the earth's bedrock.
Finland is well underway in the construction of its Onkalo high-level nuclear waste repository , which they began building in 2004 with plans to seal their facility by the end of the 21st century.
The technological method that Finland and Sweden plan to use in their permanent repositories is referred to as KBS-3 storage . In this method, spent nuclear fuel is encased in cast iron, which is then placed inside copper canisters, which are then surrounded by clay and bedrock approximately 500 meters below ground. The same or similar methods are being considered by other countries, such as the United Kingdom.
Sweden and Finland have described KBS-3 as a world-first nuclear-waste management solution. It is the product of decades of scientific research and negotiation with stakeholders, in particular with the communities that will eventually live near the buried waste.
Critical questions remain about the storage method, however. There have been widely publicized concerns in Sweden about the corrosion of test copper canisters after just a few decades. This is worrying, to say the least, because it's based on a principle of passive safety. The storage sites will be constructed, the canisters filled and sealed, and then everything will be left in the ground without any human monitoring its safe functioning and with no technological option for retrieving it. Yet, over 100,000 years the prospect of human or non-human intrusion into the site—both accidental or intentional—remains a serious threat.
The Key Information File
Another major problem is how to communicate the presence of buried nuclear waste to future generations. If spent fuel remains dangerous for 100,000 years, then clearly this is a time frame where languages can disappear and where the existence of humanity cannot be guaranteed. Transferring information about these sites into the future is a sizeable task that demands expertise and collaboration internationally across the social sciences and sciences into practices of nuclear waste memory transfer—what we refer to as nuclear memory communication .
In a project commissioned by the Swedish Nuclear Waste Management Company (SKB), we take up this precise task by writing the "Key Information File"—a document aimed at non-expert readers containing only the most essential information about Sweden's nuclear waste repository under development.
The Key Information File has been formulated as a summary document that would help future readers understand the dangers posed by buried waste. Its purpose is to guide the reader to where they can find more detailed information about the repository—acting as a "key" to other archives and forms of nuclear memory communication until the site's closure at the end of the 21st century. What happens to the Key Information File after this time is undecided, yet communicating the information that it contains to future generations is crucial.
The Key Information File we will publish in 2024 is intended to be securely stored at the entrance to the nuclear waste repository in Sweden, as well as at the National Archives in Stockholm. To ensure its durability and survival through time, the plan is for it to be reproduced in different media formats and translated into multiple languages . The initial version is in English and, when finalized, it will be translated into Swedish and other languages that have yet to be decided.
Our aim is for the file to be updated every 10 years to ensure that essential information is correct and that it remains understandable to a wide audience. We also see the need for the file to be incorporated into other intergenerational practices of knowledge transfer in the future—from its inclusion into educational syllabi in schools, to the use of graphic design and artwork to make the document distinctive and memorable, to the formation of international networks of Key Information File writing and storage in countries where, at the time of writing, decisions have not yet been made about how to store highly radioactive long-lived nuclear waste.
Fragility and short-termism: a great irony
In the process of writing the Key Information File, we have discovered many issues surrounding the efficacy of these strategies for communicating memory of nuclear waste repositories into the future. One is the remarkable fragility of programs and institutions—on more than one occasion in recent years, it has taken just one person to retire from a nuclear organization for the knowledge of an entire program of memory communication to be halted or even lost.
And if it is difficult to preserve and communicate crucial information even in the short term, what chance do we have over 100,000 years?
International attention is increasingly fixated on "impactful" short-term responses to environmental problems—usually limited to the lifespan of two or three future generations of human life. Yet the nature of long-lived nuclear waste requires us to imagine and care for a future well beyond that time horizon, and perhaps even beyond the existence of humanity.
Responding to these challenges, even partially, requires governments and research funders internationally to provide the capacity for long-term intergenerational research on these and related issues. It also demands care in developing succession plans for retiring experts to ensure their institutional knowledge and expertise is not lost. In Sweden, this could also mean committing long-term funding from the Swedish nuclear waste fund so that not only future technical problems with the waste deposition are tackled, but also future societal problems of memory and information transfer can be addressed by people with appropriate capacity and expertise.
Provided by The Conversation
Explore further
Feedback to editors

US Moon lander 'permanently' asleep after historic landing: Company

Geomagnetic storm from a solar flare could disrupt radio communications and create a striking aurora

NASA touts space research in anti-cancer fight
14 hours ago

Beyond cloning: Harnessing the power of virtual quantum broadcasting
21 hours ago

Prestigious journals make it hard for scientists who don't speak English to get published, study finds
Mar 23, 2024

Scientists develop ultra-thin semiconductor fibers that turn fabrics into wearable electronics

Saturday Citations: An anemic galaxy and a black hole with no influence. Also: A really cute bug

Research team proposes a novel type of acoustic crystal with smooth, continuous changes in elastic properties

New findings shed light on finding valuable 'green' metals

No 'human era' in Earth's geological history, scientists say
Mar 22, 2024
Relevant PhysicsForums posts
Unlocking the secrets of prof. verschure's rosetta stones.
Mar 21, 2024
Iceland warming up again - quakes swarming
Higher chance to get lightning strike by large power consumption.
Mar 20, 2024
A very puzzling rock or a pallasite / mesmosiderite or a nothing burger
Mar 16, 2024
Earth's earliest forest discovered in SW England
Mar 8, 2024
La Cumbre volcano eruption, Fernandina, Galapagos Islands
Mar 4, 2024
More from Earth Sciences
Related Stories

Finland's nuclear catacombs nearly ready to house waste
Jun 6, 2023

New glass-ceramic composite shows promise for safer storage of nuclear waste
Feb 9, 2024

Current model for storing nuclear waste is incomplete
Jan 27, 2020

Their mission: Warning future humanity about buried nuclear waste
Oct 25, 2022
Proposal advances to store nuclear waste in New Mexico
Mar 2, 2018

Measuring neutrons to reduce nuclear waste: New technique paves the way for improved nuclear waste treatment facilities
Feb 16, 2024
Recommended for you

Unintentional generation of PCBs may be producing more of the chemicals than before ban

Study reports enormous ice loss from Greenland glacier

Scientists explore complex pattern of tipping points in the Atlantic's current system

Icefields in South America are larger than all glaciers in the European Alps together

Study suggests millions are at risk using high arsenic water for cooking
Let us know if there is a problem with our content.
Use this form if you have come across a typo, inaccuracy or would like to send an edit request for the content on this page. For general inquiries, please use our contact form . For general feedback, use the public comments section below (please adhere to guidelines ).
Please select the most appropriate category to facilitate processing of your request
Thank you for taking time to provide your feedback to the editors.
Your feedback is important to us. However, we do not guarantee individual replies due to the high volume of messages.
E-mail the story
Your email address is used only to let the recipient know who sent the email. Neither your address nor the recipient's address will be used for any other purpose. The information you enter will appear in your e-mail message and is not retained by Phys.org in any form.
Newsletter sign up
Get weekly and/or daily updates delivered to your inbox. You can unsubscribe at any time and we'll never share your details to third parties.
More information Privacy policy
Donate and enjoy an ad-free experience
We keep our content available to everyone. Consider supporting Science X's mission by getting a premium account.
E-mail newsletter

An official website of the United States government
Here’s how you know
Official websites use .gov A .gov website belongs to an official government organization in the United States.
Secure .gov websites use HTTPS A lock ( Lock A locked padlock ) or https:// means you’ve safely connected to the .gov website. Share sensitive information only on official, secure websites.
https://www.nist.gov/publications/methods-maximize-detector-count-rates-small-angle-neutron-scattering-diffractometers
Methods to maximize detector count rates on small-angle neutron scattering diffractometers at reactor sources: II. Optimizing sample, source and detector sizes
Download paper, additional citation formats.
- Google Scholar
- POWER Plant ID
- POWER Events
- Connected Plant
- Distributed Energy
- International
- COVID-19 Coverage
- Carbon Capture
- Climate change
- Cybersecurity
- Distributed Power
- Electric Vehicles
- Energy Storage
- Environmental
- Instrumentation & Controls
- Legal & Regulatory
- Legislative
- Ocean/Marine
- Physical security
- Plant Design
- Power Demand
- Research and Development
- Supply Chains
- Tidal Power
- Waste to Energy
- About POWER
- Privacy Policy
- Diversity, Equity, Inclusion & Belonging
- Accessibility Statement
Bill Gates' TerraPower Ready to Build New U.S. Nuclear Power Plant
A group established by Microsoft founder Bill Gates is preparing to begin construction of a new generation of nuclear power plants in June, according to the company’s chief executive.
Chris Levesque, CEO of TerraPower, on March 18 said the company is ready to move forward with work on its Natrium reactor at the site of a former coal-fired power plant in Kemmerer, Wyoming. The group has received about $1 billion in private funding, with the U.S. government reportedly supporting the Wyoming project with as much as $2 billion.
Levesque told the Financial Times newspaper that construction work for the power plant would start in June, even if the company has not received a permit from the U.S. Nuclear Regulatory Commission (NRC). Levesque said much of the early work would not be related to nuclear activity, but rather to the design of the reactor. He said TerraPower hopes to have the plant enter commercial operation in 2030.
Liquid Sodium as Coolant
The Natrium reactor design will use liquid sodium as a coolant instead of water. Levesque told the Financial Times , “When you use liquid sodium as a coolant instead of water it’s a game-changer. Natrium plants will cost half of what light water reactor plants cost . . . and we are moving our project along pretty aggressively.”
Levesque has said the high boiling point of almost 900C for the liquid metal would bring major cost savings compared with using water.
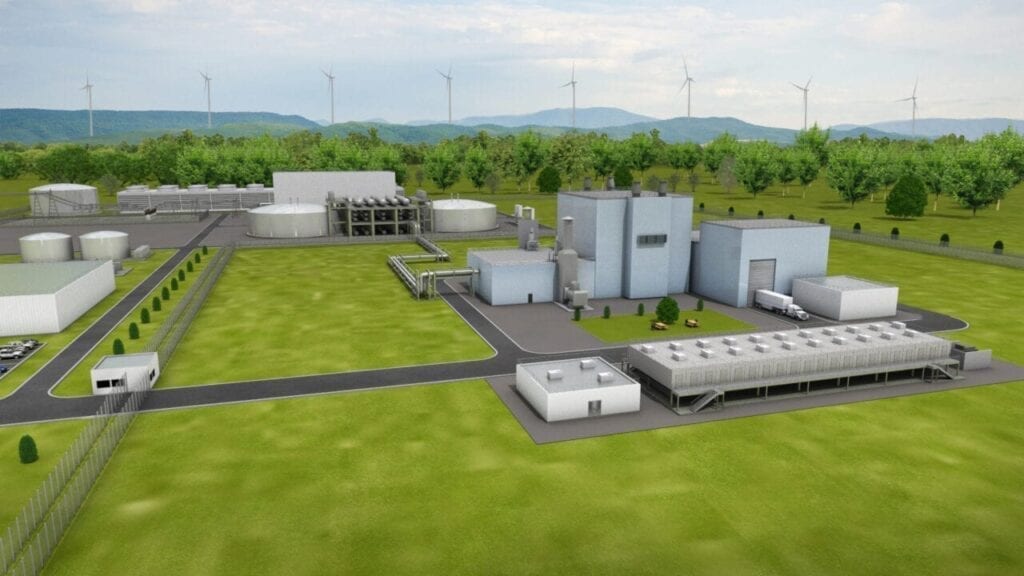
U.S. utility Southern Co. in November of last year said it had agreed to design and build an advanced demonstration nuclear reactor at Idaho National Laboratory (INL) using technology developed by TerraPower. Southern said it would build a molten-chloride reactor at INL. The U.S. Dept. of Energy (DOE) has said it would provide a five-year grant to cover 80% of the $170 million project at INL, with the rest coming from Southern, TerraPower, and other investors.
Southern Co. and TerraPower were awarded about $40 million from DOE through a public-private partnership in 2015. The money was earmarked for construction of integrated infrastructure necessary to support early development of molten chloride fast reactor, or MCFR, technology.
TerraPower in December of last year signed an agreement with Emirates Nuclear Energy Corp. That deal is designed for TerraPower to explore not only the use of its Natrium reactors to generate electricity in the United Arab Emirates, but also to produce hydrogen.
Small Reactors
TerraPower is among several groups working on a new generation of small nuclear reactors, including micro-reactors and small modular reactors (SMRs). Most of the SMRs in development would have a generation capacity of 300 MW or less. Most newer utility-scale reactors can generate 1,000 MW of power or more, such as the two units that recently entered commercial operation at Plant Vogtle in Georgia as part of an expansion there.
Energy analysts have told POWER that large-scale deployment of SMRs faces several challenges, both economic and regulatory as well as technical. Most are in agreement that government support will be needed to support new projects.
An example of the challenges is provided by Oregon-based NuScale. The company was dealt a blow to its operations with the November 2023 cancellation of a flagship project the group had planned with Utah Associated Municipal Power Systems. NuScale, founded in 2007, was the first U.S.-based SMR maker with NRC approval for its technology. The company reduced its workforce earlier this year as part of a restructuring.
There are just two SMRs deployed to date worldwide . Russia has a floating power plant, using a KLT-40S, a pressurized water reactor developed in that country. Those units are on board the Akademik Lomonosov , which is the world’s first purpose-built floating nuclear power plant.
China National Nuclear Corp. in December of last year said it had started commercial operation of the high-temperature gas-cooled modular pebble bed (HTR-PM) reactor demonstrator. The HTR-PM project is located in Rongcheng, Shandong Province, about midway between Beijing and Shanghai in eastern China.
The HTR-PM is touted as the world’s first commercially operational modular nuclear power plant with fourth-generation nuclear technology.
Demonstration Project
TerraPower has said the Kemmerer reactor would serve as a demonstration project, eventually serving as a full-scale commercial facility once complete. TerraPower and utility partner PacifiCorp, which is part of Warren Buffett’s Berkshire Hathaway, in October 2022 said the companies could deploy as many as five Natrium reactors by 2035 , if the technology proves feasible.
The Natrium reactor, which has been developed by TerraPower along with GE Hitachi Nuclear Energy , is a bit larger than most SMRs, with a generation capacity of 345 MW. The reactor also includes a molten salt-based energy storage system (ESS). TerraPower has said the ESS could increase the power output to 500 MW for a period of more than five-and-a-half hours if needed.
Levesque said, “We’re storing the heat in big tanks of molten salt, like solar plants, and that’s a big thermal battery. A plant like Natrium that can just flex very quickly with that built-in energy storage makes it very valuable.”
— Darrell Proctor is a senior associate editor for POWER ( @POWERmagazine ).
SHARE this article

IMAGES
VIDEO
COMMENTS
This paper reviews the smallness, modularity and reactor-design aspects of emerging small modular reactors (SMRs). ... An example of this is the Fixed Bed Nuclear Reactor (FBNR), a PWR 218 MWt, ... SMRs more familiar and less mysterious to people, hopefully increasing their social acceptability in the same manner research reactors were part of ...
NEICA supports the deployment of advanced reactors and develops research reactors based on fast neutrons to test the fuel and materials of advanced reactors; NELA aims to advance the research and development of advanced nuclear reactor technology and consolidate the American global leadership position in the field of civilian nuclear energy [12 ...
International Journal of Advanced Nuclear Reactor Design and Technology is a peer-reviewed, open access journal dedicated to creating a platform for sharing and exchanging the latest research results for the peaceful use of nuclear energy. The Editors welcome high-quality original research articles, technical notes, and review articles involving all aspects of nuclear science and technology.
Research reactors are nuclear reactors that are used for various scientific and technological purposes, such as producing radioisotopes, testing materials, and training personnel. This document provides an overview of the current status and future prospects of research reactors, including their types, applications, challenges, and opportunities. It also discusses the role of the IAEA in ...
reactor) in the 1970s, during the research and de velopment project of a nuclear reactor for use in submarines. The result was the PWR design named CNP-300, 1-loop PWR, and 300 MWe electrical
Much current research focuses on development of safe, competitive, and sustainable nuclear energy technologies in a post Fukushima world. This special issue of Energies focuses on advances with the potential to transform contemporary reactor technologies or advanced nuclear energy systems and fuel cycles. Technologies considered in this special ...
The reactor core used in this AI design optimization is based on a simplification of the design of the actual TCR core. The core is a right cylinder that is 1 m in diameter and 80 cm tall. Nine ...
Nuclear energy is presented as a real option in the face of the current problem of climate change and the need to reduce CO2 emissions. The nuclear reactor design with the greatest global impact throughout history and which has the most ambitious development plans is the Pressurized Water Reactor (PWR). Thus, a global review of such a reactor design is presented in this paper, utilizing the ...
On the other hand, the efficiency of the Small Modular Reactor (SMR) of the Nuclear Power Plant is 33.4% and which can be increased to 35.5%, 37.4% and 45% by using SMR with Gas Burner, SMR with ...
The purpose of this paper is to present a model for nuclear safety management in an operational organization of a nuclear power plant, based on the HTOE (human, technology, organization, and ...
The researches on nuclear reactor thermal-hydraulics have achieved outstanding progresses in the past decades. In recent years, basic research on multiphase flow dynamics and corresponding measurement technology, as well as preliminary research on Gen IV reactors based on experiments and simulations are attracting more and more attention. However, the inside complicated physics and outside ...
reactors, but its capacity has been nearly flat for the past three decades.1 High capital costs, low electricity demand growth, and competition from cheaper sources of electricity, such as natural gas and renewables, have dampened the demand for new nuclear power plants and led to the permanent shutdown of existing reactors. Thirteen nuclear ...
sion research should consider relaxed nuclear waste design criteria, raw material availability constraints and load-following designs with pulsed operation. Keywords: fusion, nuclear, perceptions, rm resources, decarbonisation, load-following, waste, EROI Corresponding author Email address: [email protected] (T. E. G. Nicholas)
This research received no specific grant from any funding agency in the public, commercial, or not-for-profit sectors. ... 2 An MFCI takes place when nuclear reactor fuel rods interact with water after a core meltdown, causing an explosion. 3 It is unlikely that the pool would have melted even if left uncooled. It is likely, however, that it ...
Towards an enhanced safety margin for the next-generation reactors, this paper presents the state-of-the-art applications of DL algorithms for nuclear power reactor safety analysis. First, this paper presents the state-of-the-art DL algorithms commonly applied for complex system modeling. ... future research focus that could improve reactor ...
Nuclear safety research started with the Fermi reactor, where multiple redundant safety systems played important roles in keeping the whole operation under control. However, in the unexpected second nuclear era, the concept of nuclear safety has gone far beyond technology, as nuclear safety has been recognized as a prevalent social issue more ...
Research on the fourth generation reactors is needed for the realisation of this development. For the fast nuclear reactors, a substantial research and development effort is required in many fields—from material sciences to safety demonstration—to attain the envisaged goals. Fusion provides a long-term vision for an efficient energy production.
This publication presents an overview of research reactor capabilities and capacities in the development of fuels and materials for innovative nuclear reactors, such as GenIV reactors. The compendium provides comprehensive information on the potential for materials and fuel testing research of 30 research reactors, both operational and in ...
Reactor Optimization Benchmark by Reinforced Learning Deborah Schwarcz1,∗, Nadav Schneider1 ,3 ∗∗, Gal Oren2 ,4 ∗∗∗, and Uri Steinitz1,∗∗∗∗ 1Soreq Nuclear Research Center, Israel 2Scientific Computing Center, Nuclear Research Center - Negev, Israel 3Department of Electrical & Computer Engineering, Ben-Gurion University, Israel 4Department of Computer Science, Technion ...
Since the early days of its inception, in 1957, the IAEA has supported nuclear fusion research. The IAEA Department of Nuclear Sciences and Applications and the Department of Nuclear Energy ... can be harvested for powering the reactor and producing electricity, respectively. 7 Tapping the Energy At the core of the stars, fusion reactions ...
Unlike other research reactors, Fast Breeder Test Reactor (FBTR) is an SFR and has a steam production circuit with a turbine generator for producing electricity. It is representative of a nuclear power plant and has given the confidence in developing a prototype fast breeder reactor. 4.2 Choice of nuclear power reactor type for India
The grand total of lives lost from all nuclear power generation to date, while hard to quantify, is certainly far lower than the number of people killed by air pollution related to the burning of fossil fuels; a recent paper by NASA scientists concluded that nuclear power saved roughly 1.8 million lives from 1971 to 2009 thanks to avoided air ...
Nuclear power plants produce three kinds of radioactive waste:. Short-lived low- and intermediate-level waste; Long-lived low- and intermediate-level waste; Long-lived and highly radioactive waste ...
Research paper. A historical review and analysis on the selection of nuclear reactor types and implications to development programs for advanced reactors; A Japanese study ... In recent years, the development of innovative nuclear reactor technologies has been widely advocated in the United States and other leading countries, with governments ...
Abstract Methods for maximizing the detector count rate for the same scattering angle range and resolution are related to choosing the optimum pinhole collimation parameters which include the source and sample aperture sizes and collimation lengths on small-angle neutron scattering diffractometers located at reactor neutron sources.
In a paper published by the Chinese Academy of Sciences ' peer-reviewed journal Scientia Sinica Technologica, the research team said its prototype lithium-cooled nuclear reactor system has ...
A nuclear fission reactor harnesses a nuclear chain reaction, normally involving uranium-238. There are a variety of different fusion reactors in use, including boiling water reactors, pressurized ...
The Natrium reactor, which has been developed by TerraPower along with GE Hitachi Nuclear Energy, is a bit larger than most SMRs, with a generation capacity of 345 MW. The reactor also includes a ...
Short research papers should be submitted to the journal Annals of Nuclear Energy. PNE reserves the right to reject papers which are based solely on routine application of computer codes used to produce reactor designs or reproduce known reactor phenomena. Such papers, although worthy, are best left as laboratory reports.
The world's long-running $20bn ITER research project, a consortium of the US, Japan, Europe, China, and Russia, looks ever more like a beached whale in this contest.